100 Star Formation
Andrew Franknoi; David Morrison; and Sidney C. Wolff
Learning Objectives
By the end of this section, you will be able to:
- Identify the sometimes-violent processes by which parts of a molecular cloud collapse to produce stars
- Recognize some of the structures seen in images of molecular clouds like the one in Orion
- Explain how the environment of a molecular cloud enables the formation of stars
- Describe how advancing waves of star formation cause a molecular cloud to evolve
As we begin our exploration of how stars are formed, let’s review some basics about stars discussed in earlier chapters:
- Stable (main-sequence) stars such as our Sun maintain equilibrium by producing energy through nuclear fusion in their cores. The ability to generate energy by fusion defines a star.
- Each second in the Sun, approximately 600 million tons of hydrogen undergo fusion into helium, with about 4 million tons turning into energy in the process. This rate of hydrogen use means that eventually the Sun (and all other stars) will run out of central fuel.
- Stars come with many different masses, ranging from 1/12 solar masses (MSun) to roughly 100–200 MSun. There are far more low-mass than high-mass stars.
- The most massive main-sequence stars (spectral type O) are also the most luminous and have the highest surface temperature. The lowest-mass stars on the main sequence (spectral type M or L) are the least luminous and the coolest.
- A galaxy of stars such as the Milky Way contains enormous amounts of gas and dust—enough to make billions of stars like the Sun.
If we want to find stars still in the process of formation, we must look in places that have plenty of the raw material from which stars are assembled. Since stars are made of gas, we focus our attention (and our telescopes) on the dense and cold clouds of gas and dust that dot the Milky Way (see Figure 1).
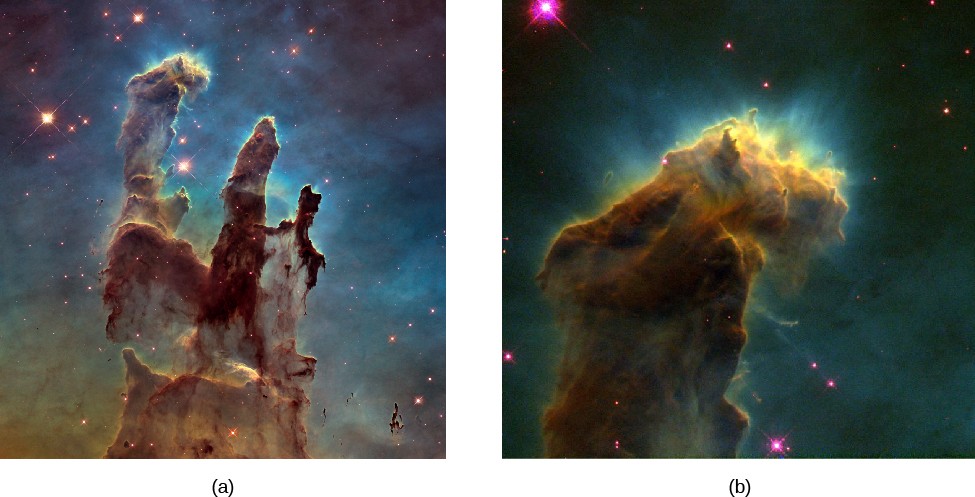
Molecular Clouds: Stellar Nurseries
As we saw in Between the Stars: Gas and Dust in Space, the most massive reservoirs of interstellar matter—and some of the most massive objects in the Milky Way Galaxy—are the giant molecular clouds. These clouds have cold interiors with characteristic temperatures of only 10–20 K; most of their gas atoms are bound into molecules. These clouds turn out to be the birthplaces of most stars in our Galaxy.
The masses of molecular clouds range from a thousand times the mass of the Sun to about 3 million solar masses. Molecular clouds have a complex filamentary structure, similar to cirrus clouds in Earth’s atmosphere, but much less dense. The molecular cloud filaments can be up to 1000 light-years long. Within the clouds are cold, dense regions with typical masses of 50 to 500 times the mass of the Sun; we give these regions the highly technical name clumps. Within these clumps, there are even denser, smaller regions called cores. The cores are the embryos of stars. The conditions in these cores—low temperature and high density—are just what is required to make stars. Remember that the essence of the life story of any star is the ongoing competition between two forces: gravity and pressure. The force of gravity, pulling inward, tries to make a star collapse. Internal pressure produced by the motions of the gas atoms, pushing outward, tries to force the star to expand. When a star is first forming, low temperature (and hence, low pressure) and high density (hence, greater gravitational attraction) both work to give gravity the advantage. In order to form a star—that is, a dense, hot ball of matter capable of starting nuclear reactions deep within—we need a typical core of interstellar atoms and molecules to shrink in radius and increase in density by a factor of nearly 1020. It is the force of gravity that produces this drastic collapse.
The Orion Molecular Cloud
Let’s discuss what happens in regions of star formation by considering a nearby site where stars are forming right now. One of the best-studied stellar nurseries is in the constellation of Orion, The Hunter, about 1500 light-years away (Figure 2). The pattern of the hunter is easy to recognize by the conspicuous “belt” of three stars that mark his waist. The Orion molecular cloud is much larger than the star pattern and is truly an impressive structure. In its long dimension, it stretches over a distance of about 100 light-years. The total quantity of molecular gas is about 200,000 times the mass of the Sun. Most of the cloud does not glow with visible light but betrays its presence by the radiation that the dusty gas gives off at infrared and radio wavelengths.
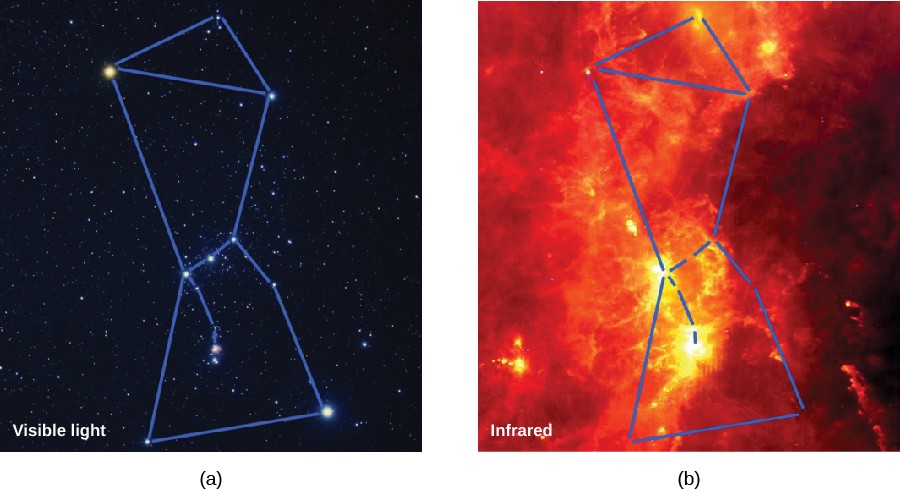
The stars in Orion’s belt are typically about 5 million years old, whereas the stars near the middle of the “sword” hanging from Orion’s belt are only 300,000 to 1 million years old. The region about halfway down the sword where star formation is still taking place is called the Orion Nebula. About 2200 young stars are found in this region, which is only slightly larger than a dozen light-years in diameter. The Orion Nebula also contains a tight cluster of stars called the Trapezium (Figure 4). The brightest Trapezium stars can be seen easily with a small telescope.
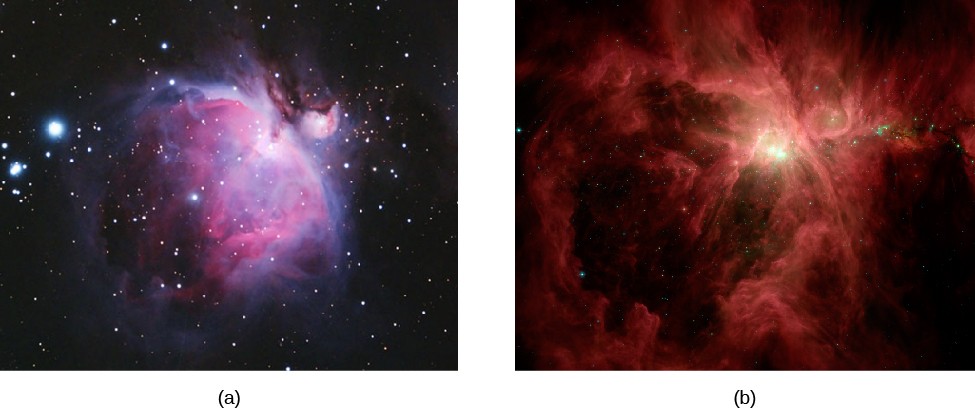
Compare this with our own solar neighborhood, where the typical spacing between stars is about 3 light-years. Only a small number of stars in the Orion cluster can be seen with visible light, but infrared images—which penetrate the dust better—detect the more than 2000 stars that are part of the group (Figure 4).
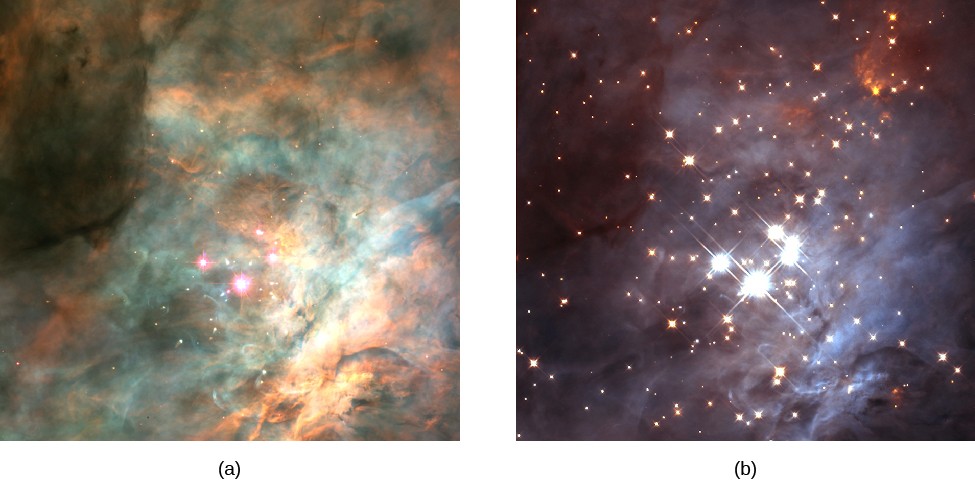
Studies of Orion and other star-forming regions show that star formation is not a very efficient process. In the region of the Orion Nebula, about 1% of the material in the cloud has been turned into stars. That is why we still see a substantial amount of gas and dust near the Trapezium stars. The leftover material is eventually heated, either by the radiation and winds from the hot stars that form or by explosions of the most massive stars. (We will see in later chapters that the most massive stars go through their lives very quickly and end by exploding.)
Take a journey through the Orion Nebula to view a nice narrated video tour of this region.
Whether gently or explosively, the material in the neighborhood of the new stars is blown away into interstellar space. Older groups or clusters of stars can now be easily observed in visible light because they are no longer shrouded in dust and gas (Figure 5).
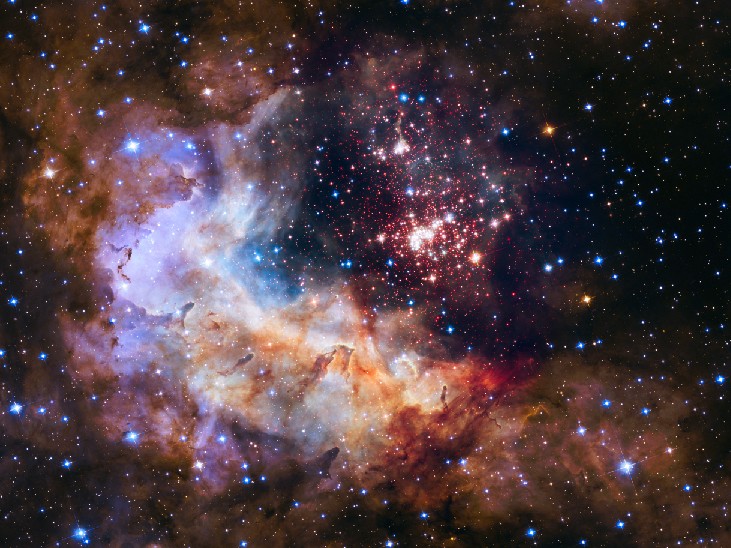
Although we do not know what initially caused stars to begin forming in Orion, there is good evidence that the first generation of stars triggered the formation of additional stars, which in turn led to the formation of still more stars (Figure 6).
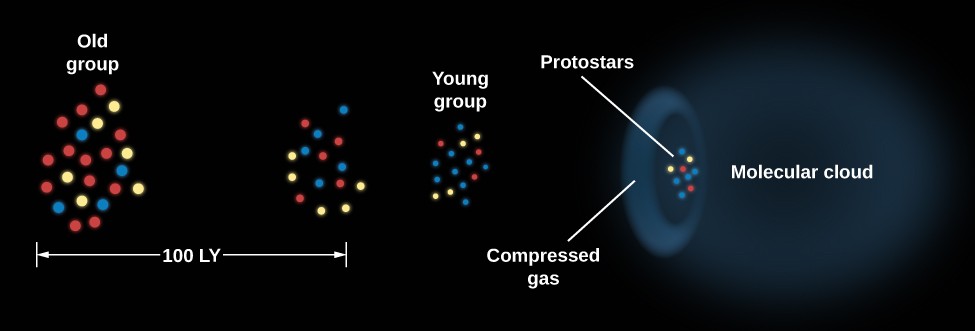
The basic idea of triggered star formation is this: when a massive star is formed, it emits a large amount of ultraviolet radiation and ejects high-speed gas in the form of a stellar wind. This injection of energy heats the gas around the stars and causes it to expand. When massive stars exhaust their supply of fuel, they explode, and the energy of the explosion also heats the gas. The hot gases pile into the surrounding cold molecular cloud, compressing the material in it and increasing its density. If this increase in density is large enough, gravity will overcome pressure, and stars will begin to form in the compressed gas. Such a chain reaction—where the brightest and hottest stars of one area become the cause of star formation “next door”—seems to have occurred not only in Orion but also in many other molecular clouds.
There are many molecular clouds that form only (or mainly) low-mass stars. Because low-mass stars do not have strong winds and do not die by exploding, triggered star formation cannot occur in these clouds. There are also stars that form in relative isolation in small cores. Therefore, not all star formation is originally triggered by the death of massive stars. However, there are likely to be other possible triggers, such as spiral density waves and other processes we do not yet understand.
The Birth of a Star
Although regions such as Orion give us clues about how star formation begins, the subsequent stages are still shrouded in mystery (and a lot of dust). There is an enormous difference between the density of a molecular cloud core and the density of the youngest stars that can be detected. Direct observations of this collapse to higher density are nearly impossible for two reasons. First, the dust-shrouded interiors of molecular clouds where stellar births take place cannot be observed with visible light. Second, the timescale for the initial collapse—thousands of years—is very short, astronomically speaking. Since each star spends such a tiny fraction of its life in this stage, relatively few stars are going through the collapse process at any given time. Nevertheless, through a combination of theoretical calculations and the limited observations available, astronomers have pieced together a picture of what the earliest stages of stellar evolution are likely to be.
The first step in the process of creating stars is the formation of dense cores within a clump of gas and dust (Figure 7(a)). It is generally thought that all the material for the star comes from the core, the larger structure surrounding the forming star. Eventually, the gravitational force of the infalling gas becomes strong enough to overwhelm the pressure exerted by the cold material that forms the dense cores. The material then undergoes a rapid collapse, and the density of the core increases greatly as a result. During the time a dense core is contracting to become a true star, but before the fusion of protons to produce helium begins, we call the object a protostar.
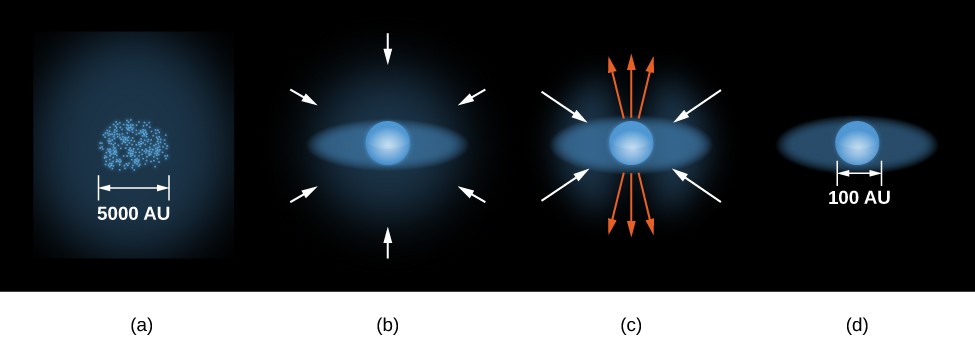
The natural turbulence inside a clump tends to give any portion of it some initial spinning motion (even if it is very slow). As a result, each collapsing core is expected to spin. According to the law of conservation of angular momentum (discussed in the chapter on Orbits and Gravity), a rotating body spins more rapidly as it decreases in size. In other words, if the object can turn its material around a smaller circle, it can move that material more quickly—like a figure skater spinning more rapidly as she brings her arms in tight to her body. This is exactly what happens when a core contracts to form a protostar: as it shrinks, its rate of spin increases.
But all directions on a spinning sphere are not created equal. As the protostar rotates, it is much easier for material to fall right onto the poles (which spin most slowly) than onto the equator (where material moves around most rapidly). Therefore, gas and dust falling in toward the protostar’s equator are “held back” by the rotation and form a whirling extended disk around the equator (part b in Figure 7). You may have observed this same “equator effect” on the amusement park ride in which you stand with your back to a cylinder that is spun faster and faster. As you spin really fast, you are pushed against the wall so strongly that you cannot possibly fall toward the center of the cylinder. Gas can, however, fall onto the protostar easily from directions away from the star’s equator.
The protostar and disk at this stage are embedded in an envelope of dust and gas from which material is still falling onto the protostar. This dusty envelope blocks visible light, but infrared radiation can get through. As a result, in this phase of its evolution, the protostar itself is emitting infrared radiation and so is observable only in the infrared region of the spectrum. Once almost all of the available material has been accreted and the central protostar has reached nearly its final mass, it is given a special name: it is called a T Tauri star, named after one of the best studied and brightest members of this class of stars, which was discovered in the constellation of Taurus. (Astronomers have a tendency to name types of stars after the first example they discover or come to understand. It’s not an elegant system, but it works.) Only stars with masses less than or similar to the mass of the Sun become T Tauri stars. Massive stars do not go through this stage, although they do appear to follow the formation scenario illustrated in Figure 7.
Winds and Jets
Recent observations suggest that T Tauri stars may actually be stars in a middle stage between protostars and hydrogen-fusing stars such as the Sun. High-resolution infrared images have revealed jets of material as well as stellar winds coming from some T Tauri stars, proof of interaction with their environment. A stellar wind consists mainly of protons (hydrogen nuclei) and electrons streaming away from the star at speeds of a few hundred kilometers per second (several hundred thousand miles per hour). When the wind first starts up, the disk of material around the star’s equator blocks the wind in its direction. Where the wind particles can escape most effectively is in the direction of the star’s poles.
Astronomers have actually seen evidence of these beams of particles shooting out in opposite directions from the popular regions of newly formed stars. In many cases, these beams point back to the location of a protostar that is still so completely shrouded in dust that we cannot yet see it (Figure 8).
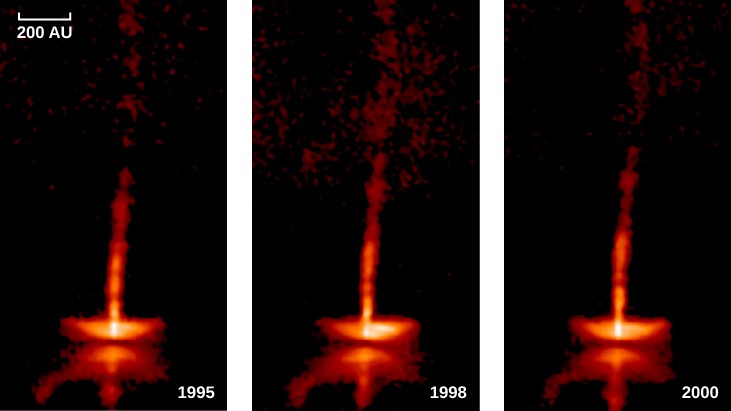
On occasion, the jets of high-speed particles streaming away from the protostar collide with a somewhat-denser lump of gas nearby, excite its atoms, and cause them to emit light. These glowing regions, each of which is known as a Herbig-Haro (HH) object after the two astronomers who first identified them, allow us to trace the progress of the jet to a distance of a light-year or more from the star that produced it. Figure 9 shows two spectacular images of HH objects.
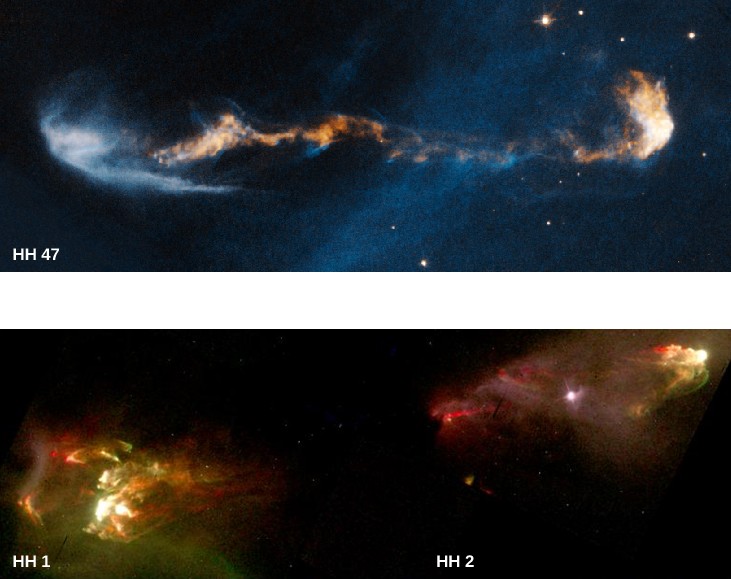
The wind from a forming star will ultimately sweep away the material that remains in the obscuring envelope of dust and gas, leaving behind the naked disk and protostar, which can then be seen with visible light. We should note that at this point, the protostar itself is still contracting slowly and has not yet reached the main-sequence stage on the H–R diagram (a concept introduced in the chapter The Stars: A Celestial Census). The disk can be detected directly when observed at infrared wavelengths or when it is seen silhouetted against a bright background (Figure 10).
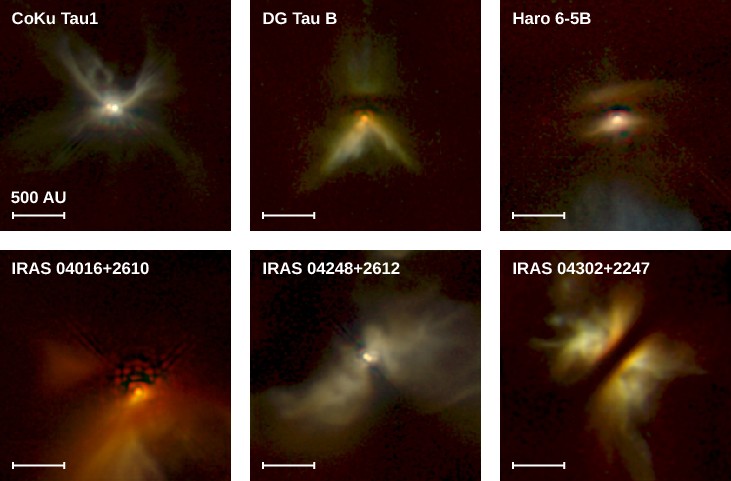
This description of a protostar surrounded by a rotating disk of gas and dust sounds very much like what happened in our solar system when the Sun and planets formed. Indeed, one of the most important discoveries from the study of star formation in the last decade of the twentieth century was that disks are an inevitable byproduct of the process of creating stars. The next questions that astronomers set out to answer was: will the disks around protostars also form planets? And if so, how often? We will return to these questions later in this chapter.
To keep things simple, we have described the formation of single stars. Many stars, however, are members of binary or triple systems, where several stars are born together. In this case, the stars form in nearly the same way. Widely separated binaries may each have their own disk; close binaries may share a single disk.
Key concepts and summary
Most stars form in giant molecular clouds with masses as large as 3 × 106 solar masses. The most well-studied molecular cloud is Orion, where star formation is currently taking place. Molecular clouds typically contain regions of higher density called clumps, which in turn contain several even-denser cores of gas and dust, each of which may become a star. A star can form inside a core if its density is high enough that gravity can overwhelm the internal pressure and cause the gas and dust to collapse. The accumulation of material halts when a protostar develops a strong stellar wind, leading to jets of material being observed coming from the star. These jets of material can collide with the material around the star and produce regions that emit light that are known as Herbig-Haro objects.
Glossary
giant molecular clouds: large, cold interstellar clouds with diameters of dozens of light-years and typical masses of 105 solar masses; found in the spiral arms of galaxies, these clouds are where stars form
Herbig-Haro (HH) object: luminous knots of gas in an area of star formation that are set to glow by jets of material from a protostar
protostar: a very young star still in the process of formation, before nuclear fusion begins
stellar wind: the outflow of gas, sometimes at speeds as high as hundreds of kilometers per second, from a star