11 Mechanisms of Evolution
Learning Objectives
After studying this chapter, you should be able to:
- Describe how natural selection, mutation, genetic drift, and gene flow lead to evolution
- Differentiate between divergent evolution and convergent evolution
- Describe the definition of species and how species are identified as being different
- Explain the difference between allopatric and sympatric speciation
Natural Selection
As you will recall, evolution refers to a change in the characteristics of a population over time. More specifically, evolution is caused by changes in the alleles present in a population. Alleles are the alternative forms of genes present in an organism, which are inherited through sexual or asexual reproduction. For example, the gene for hair color in humans has several different alleles, which will lead to variations such as brown, red, black, or blond hair. The alleles present in an organism are known as the genotype, while the phenotype refers to the physical characteristics that result from expression of the genotype. In this chapter, you will learn about several mechanisms that can change the alleles present in the organisms that make up a population. You will also learn how changes in allele frequencies can lead to the formation of new species over time.
As discussed in a previous chapter, natural selection is the main way in which populations evolve. Charles Darwin and Alfred Russell Wallace noticed that there is always a struggle for survival among members of a population due to the fact that resources are always limited. This means only a portion of the offspring born in every generation will survive. Those with more favorable traits for their environment are more likely to survive than those with less favorable traits. Since many of the traits present in an organism are inherited from their parents, these favorable traits are then more likely to be passed on to future generations. Organisms that inherit the more beneficial traits from their parents are said to have increased fitness, or a better chance of surviving and reproducing.
Demonstrations of evolution by natural selection in the real world can be time-consuming. One of the best demonstrations has been in the very birds that helped to inspire the theory, the Galápagos finches. Peter and Rosemary Grant and their colleagues have studied Galápagos finch populations every year since 1976 and have provided important demonstrations of the operation of natural selection. The Grants found changes from one generation to the next in the beak shapes of the medium ground finches on the Galápagos island of Daphne Major. The medium ground finch feeds on seeds. The birds have inherited variation in the beak shape with some individuals having wide, deep beaks and others having thinner beaks. Large-beaked birds feed more efficiently on large, hard seeds, whereas smaller-beaked birds feed more efficiently on small, soft seeds.
During 1977, a drought period altered vegetation on the island. After this period, the number of seeds declined dramatically: the decline in small, soft seeds was greater than the decline in large, hard seeds. The large-beaked birds were able to survive better than the small-beaked birds the following year. The year following the drought when the Grants measured beak sizes in the much-reduced population, they found that the average beak size was larger (Figure 1). This was clear evidence for natural selection (differences in survival) of beak size caused by the availability of seeds. The Grants had studied the inheritance of beak sizes and knew that the surviving large-beaked birds would tend to produce offspring with larger beaks, so the selection would lead to the evolution of beak size. Subsequent studies by the Grants have demonstrated selection on and evolution of beak size in this species in response to changing conditions on the island. The evolution has occurred both to larger beaks, as in this case, and to smaller beaks when large seeds became rare.
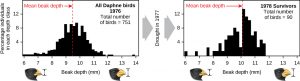
Knowledge Check
Variation and Adaptation
Natural selection can only take place if there is variation, or differences, among individuals in a population. Importantly, these differences must have some genetic basis; otherwise, selection will not lead to change in the next generation. This is critical because variation among individuals can be caused by non-genetic reasons, such as an individual being taller because of better nutrition rather than different genes.
A heritable trait that aids the survival and reproduction of an organism in its present environment is called an adaptation. An adaptation is a “match” of the organism to the environment. Adaptation to an environment comes about when a change in the range of genetic variation occurs over time that increases or maintains the match of the population with its environment. The variations in finch beaks shifted from generation to generation providing adaptation to food availability.
An adaptation is not the same as an acclimation, or a temporary change in an organism’s phenotype due to changes in the environment. For example, a dog raised in a warm environment may have a thin coat of fur, but it will gradually get thicker if the dog is moved to a colder environment. However, this temporary acclimation would not be passed on to the dog’s offspring.
Whether or not a trait is favorable depends on the environment at the time. The same traits do not always have the same relative benefit or disadvantage because environmental conditions can change. For example, finches with large beaks were benefited in one climate, while small beaks were a disadvantage; in a different climate, the relationship reversed.
Other Mechanisms of Evolution
Natural selection is considered the main mechanism that causes populations to evolve. However, there are several other mechanisms of evolution, including mutation, genetic drift, and gene flow.
Mutation
Mutation is a source of new alleles in a population, thus it leads to an increase in variation among the members of the population. Mutation is a change in the DNA sequence of the gene. An individual that has a mutated gene might have a different trait than other individuals in the population. However, this is not always the case. A mutation can have one of three outcomes on the organisms’ appearance (or phenotype):
- A negative mutation affects the phenotype of the organism in a way that gives it reduced fitness—lower likelihood of survival, resulting in fewer offspring.
- A positive mutation produces a phenotype with a beneficial effect on fitness.
- Many mutations, called neutral mutations, will have no effect on fitness.
The change in frequency resulting from mutation is small, so its effect on evolution is small unless it interacts with one of the other factors, such as selection. A mutation may produce an allele that is selected against, selected for, or selectively neutral. Harmful mutations are removed from the population by selection and will generally only be found in very low frequencies equal to the mutation rate. Beneficial mutations will spread through the population through selection, although that initial spread is slow. Whether or not a mutation is beneficial or harmful is determined by whether it helps an organism survive to sexual maturity and reproduce. It should be noted that mutation is the ultimate source of genetic variation in all populations—new alleles, and, therefore, new genetic variations arise through mutation. Mutations leading to antibiotic resistance in bacteria is a growing problem in the medical field. Because bacteria reproduce very quickly, they accumulate mutations rapidly. This can allow the bacteria to become resistant to antibiotic drugs (Figure 2).
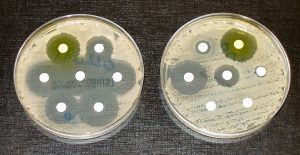
Genetic Drift
Another way a population’s allele frequencies can change is genetic drift (Figure 3), which is simply the effect of chance. Genetic drift always leads to a reduction in the variation present in a population. Genetic drift is most important in small populations. Drift would be completely absent in a population with infinite individuals, but, of course, no population is this large. Genetic drift occurs because the alleles in an offspring generation are a random sample of the alleles in the parent generation. Alleles may or may not make it into the next generation due to chance events including mortality of an individual, events affecting finding a mate, and even the events affecting which gametes (sperm and egg) end up in fertilizations. If one individual in a population of ten individuals happens to die before it leaves any offspring to the next generation, all of its genes—a tenth of the population’s gene pool—will be suddenly lost. In a population of 100, that 1 individual represents only 1 percent of the overall gene pool; therefore, it has much less impact on the population’s genetic structure and is unlikely to remove all copies of even a relatively rare allele.
Imagine a population of ten individuals, half with allele A and half with allele a. In a stable population, the next generation will also have ten individuals. Choose that generation randomly by flipping a coin ten times and let heads be A and tails be a. It is unlikely that the next generation will have exactly half of each allele. There might be six of one and four of the other, or some different set of frequencies. Thus, the allele frequencies have changed and evolution has occurred. A coin will no longer work to choose the next generation (because the odds are no longer one half for each allele). The frequency in each generation will drift up and down on what is known as a “random walk” until at one point either all A or all a are chosen and that allele is fixed from that point on. This could take a very long time for a large population. This simplification is not very biological, but it can be shown that real populations behave this way. The effect of drift on frequencies is greater the smaller a population is. Its effect is also greater on an allele with a frequency far from one half. Drift will influence every allele, even those that are being naturally selected.
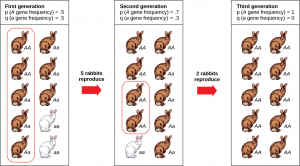
Genetic drift can also be magnified by natural or human-caused events, such as a disaster that randomly kills a large portion of the population, which is known as the bottleneck effect that results in a large portion of the genome suddenly being wiped out (Figure 4). In one fell swoop, the genetic structure of the survivors becomes the genetic structure of the entire population, which may be very different from the pre-disaster population. The disaster must be one that kills for reasons unrelated to the organism’s traits, such as a hurricane or lava flow. A mass killing caused by unusually cold temperatures at night, is likely to affect individuals differently depending on the alleles they possess that confer cold hardiness.
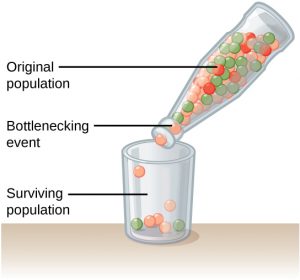
Another scenario in which populations might experience a strong influence of genetic drift is if some portion of the population leaves to start a new population in a new location, or if a population gets divided by a physical barrier of some kind. In this situation, those individuals are unlikely to be representative of the entire population which results in the founder effect. The founder effect occurs when the genetic structure matches that of the new population’s founding fathers and mothers. The founder effect is believed to have been a key factor in the genetic history of the Afrikaner population of Dutch settlers in South Africa, as evidenced by mutations that are common in Afrikaners but rare in most other populations. This is likely due to a higher-than-normal proportion of the founding colonists, which were a small sample of the original population, carried these mutations. As a result, the population expresses unusually high incidences of Huntington’s disease (HD) and Fanconi anemia (FA), a genetic disorder known to cause bone marrow and congenital abnormalities, and even cancer.
Gene Flow
Another important evolutionary force is gene flow, or the flow of alleles in and out of a population resulting from the migration of individuals (Figure 5). While some populations are fairly stable, others experience more flux. Many plants, for example, send their seeds far and wide, by wind or in the guts of animals; these seeds may introduce alleles common in the source population to a new population in which they are rare. Like mutation, gene flow leads to an increase in the variation present in a population because the migration of individuals into the population leads to the introduction of new alleles.
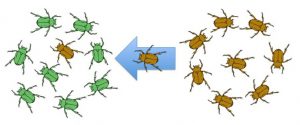
Knowledge Check
Patterns of Evolution
The evolution of species has resulted in enormous variation in form and function. When two species evolve in different directions from a common point, it is called divergent evolution. Such divergent evolution can be seen in the forms of the reproductive organs of flowering plants, which share the same basic anatomies; however, they can look very different as a result of selection in different physical environments, and adaptation to different kinds of pollinators (Figure 6).
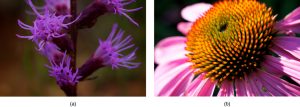
In other cases, similar phenotypes evolve independently in distantly related species. For example, flight has evolved in both bats and insects, and they both have structures we refer to as wings, which are adaptations to flight. The wings of bats and insects, however, evolved from very different original structures. When similar structures arise through evolution independently in different species it is called convergent evolution (Figure 7). The wings of bats and insects are called analogous structures; they are similar in function and appearance but do not share an origin in a common ancestor. Instead, they evolved independently in the two lineages. The wings of a hummingbird and an ostrich are homologous structures, meaning they share similarities (despite their differences resulting from evolutionary divergence). The wings of hummingbirds and ostriches did not evolve independently in the hummingbird lineage and the ostrich lineage—they descended from a common ancestor with wings.
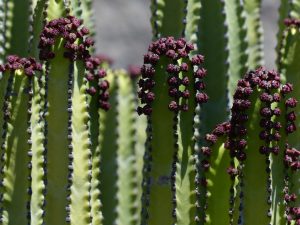
Speciation
The biological definition of species, which works for sexually reproducing organisms, is a group of actually or potentially interbreeding individuals. According to this definition, one species is distinguished from another by the possibility of matings between individuals from each species to produce fertile offspring. There are exceptions to this rule. Many species are similar enough that hybrid offspring are possible and may often occur in nature, but for the majority of species this rule generally holds. In fact, the presence of hybrids between similar species suggests that they may have descended from a single interbreeding species and that the speciation process may not yet be completed. This definition also does not work for asexually reproducing organisms like bacteria.
Given the extraordinary diversity of life on the planet there must be mechanisms for speciation: the formation of two species from one original species. Darwin envisioned this process as a branching event and diagrammed the process in the only illustration found in On the Origin of Species (Figure 8a). Biologists think of speciation events as the splitting of one ancestral species into two descendant species (Figure 8b). There is no reason why there might not be more than two species formed at one time except that it is less likely and such multiple events can also be conceptualized as single splits occurring close in time.
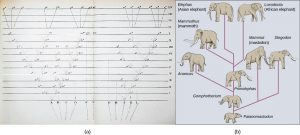
For speciation to occur, two new populations must be formed from one original population, and they must evolve in such a way that it becomes impossible for individuals from the two new populations to interbreed. Biologists have proposed mechanisms by which this could occur that fall into two broad categories. Allopatric speciation, meaning speciation in “other homelands,” involves a geographic separation of populations from a parent species and subsequent evolution. Sympatric speciation, meaning speciation in the “same homeland,” involves speciation occurring within a parent species while remaining in one location.
Allopatric Speciation
A geographically continuous population has a gene pool that is relatively homogeneous. Gene flow, the movement of alleles across the range of the species, is relatively free because individuals can move and then mate with individuals in their new location. Thus, the frequency of an allele at one end of a distribution will be similar to the frequency of the allele at the other end. When populations become geographically disconnected that free-flow of alleles is prevented. When that separation lasts for a period of time, the two populations are able to evolve along different trajectories. Thus, their allele frequencies gradually become more and more different as new alleles independently arise by mutation in each population. Typically, environmental conditions, such as climate, resources, predators, and competitors, for the two populations will differ causing natural selection to favor different sets of adaptations in each group. If enough differences accumulate over time, the two populations will no longer be able to produce viable offspring, and they will now be two distinct species.
Isolation of populations leading to allopatric speciation can occur in a variety of ways: from a river forming a new branch, erosion forming a new valley, or a group of organisms traveling to a new location without the ability to return, such as seeds floating over the ocean to an island. The nature of the geographic separation necessary to isolate populations depends entirely on the biology of the organism and its potential for dispersal. If two flying insect populations took up residence in separate nearby valleys, chances are that individuals from each population would fly back and forth, continuing gene flow. However, if two rodent populations became divided by the formation of a new lake, continued gene flow would be unlikely; therefore, speciation would be more likely.
Scientists have documented numerous cases of allopatric speciation taking place. For example, along the west coast of the United States, two separate subspecies of spotted owls exist. The northern spotted owl has genetic and phenotypic differences from its close relative, the Mexican spotted owl, which lives in the south (Figure 9). The cause of their initial separation is not clear, but it may have been caused by the glaciers of the ice age dividing an initial population into two.
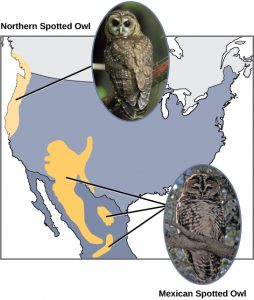
Additionally, scientists have found that the further the distance between two groups that once were the same species, the more likely for speciation to take place. This seems logical because as the distance increases, the various environmental factors would likely have less in common than locations in close proximity. Consider the two owls; in the north, the climate is cooler than in the south; the other types of organisms in each ecosystem differ, as do their behaviors and habits; also, the hunting habits and prey choices of the owls in the south vary from the northern ones. These variances can lead to evolved differences in the owls, and over time speciation will likely occur unless gene flow between the populations is restored.
In some cases, a population of one species disperses throughout an area, and each finds a distinct niche or isolated habitat. Over time, the varied demands of their new lifestyles lead to multiple speciation events originating from a single species, which is called adaptive radiation. From one point of origin, many adaptations evolve causing the species to radiate into several new ones. Island archipelagos like the Hawaiian Islands provide an ideal context for adaptive radiation events because water surrounds each island, which leads to geographical isolation for many organisms. The Hawaiian honeycreeper illustrates one example of adaptive radiation. From a single species, called the founder species, numerous species have evolved, including the eight shown in Figure 10.
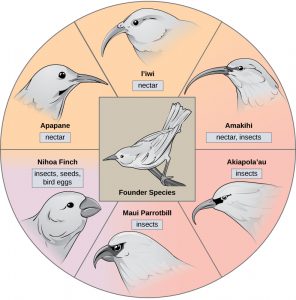
Notice the differences in the species’ beaks in Figure 10. Change in the genetic variation for beaks in response to natural selection based on specific food sources in each new habitat led to evolution of a different beak suited to the specific food source. The fruit and seed-eating birds have thicker, stronger beaks which are suited to break hard nuts. The nectar-eating birds have long beaks to dip into flowers to reach their nectar. The insect-eating birds have beaks like swords, appropriate for stabbing and impaling insects. Darwin’s finches are another well-studied example of adaptive radiation in an archipelago.
Sympatric Speciation
Can divergence occur if no physical barriers are in place to separate individuals who continue to live and reproduce in the same habitat? A number of mechanisms for sympatric speciation have been proposed and studied.
Imagine a species of fish that lived in a lake. As the population grew, competition for food also grew. Under pressure to find food, suppose that a group of these fish had the genetic flexibility to discover and feed off another resource that was unused by the other fish. What if this new food source was found at a different depth of the lake? Over time, those feeding on the second food source would interact more with each other than the other fish; therefore they would breed together as well. Offspring of these fish would likely behave as their parents and feed and live in the same area, keeping them separate from the original population. If this group of fish continued to remain separate from the first population, eventually sympatric speciation might occur as more genetic differences accumulated between them.
This scenario does play out in nature, as do others that lead to reproductive isolation. One such place is Lake Victoria in Africa, famous for its sympatric speciation of cichlid fish. Researchers have found hundreds of sympatric speciation events in these fish, which have not only happened in great number, but also over a short period of time. Figure 11 shows this type of speciation among a cichlid fish population in Nicaragua. In this locale, two types of cichlids live in the same geographic location; however, they have come to have different morphologies that allow them to eat various food sources.
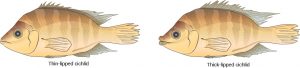
Knowledge Check
Attribution
“Evolution and Its Processes” in OpenStax Concepts of Biology, modified by Sean Whitcomb. License: CC BY
Media Attributions
- Finch_beak_graph © OpenStax is licensed under a CC BY (Attribution) license
- Antibiotic sensitivity and resistance © Graham Beards is licensed under a CC BY-SA (Attribution ShareAlike) license
- Genetic_drift © OpenStax is licensed under a CC BY (Attribution) license
- Bottleneck © OpenStax is licensed under a CC BY (Attribution) license
- Gene flow beetles © Tsaneda is licensed under a CC BY (Attribution) license
- Divergent evolution flowers © Cory Zanker adapted by OpenStax is licensed under a CC BY (Attribution) license
- Euphorbia © Hans is licensed under a Public Domain license
- Speciation of elephants © OpenStax is licensed under a CC BY (Attribution) license
- Spotted_owl_speciation © John and Karen Hollingsworth, USFWS; Bill Radke, USFWS adapted by OpenStax is licensed under a CC BY (Attribution) license
- Adaptive_radiation © OpenStax is licensed under a CC BY (Attribution) license
- Cichlids © OpenStax is licensed under a CC BY (Attribution) license