5.7 Thriving Near Volcanoes
“Every act of creation is, first of all, an act of destruction.” -Pablo Picasso
Volcanic eruptions are deadly; molten lava destroys the surrounding land and vegetation, and unpredictable lahars will bury other regions downslope of the volcano. Even if a community is fortunate to survive a violent eruption accompanied with these and other hazards such as ash, toxic gases, and pyroclastic flows, these hazards can still cause fatalities, the death of livestock, and the immeasurable destruction of infrastructure such as buildings, homes, and farmlands.
As terrible as a volcanic eruption may be for our society, we also must acknowledge that without volcanism, life as we know it may never have begun on Earth! Volcanic activity is not simply limited to eruptions of lava, gases, or ash. Most active volcanoes are continuous sources of heat and chemicals. For our earliest ancestor on the planet (which was likely bacteria), which sought its energy from heat or chemicals, areas of volcanic activity were a gold mine!
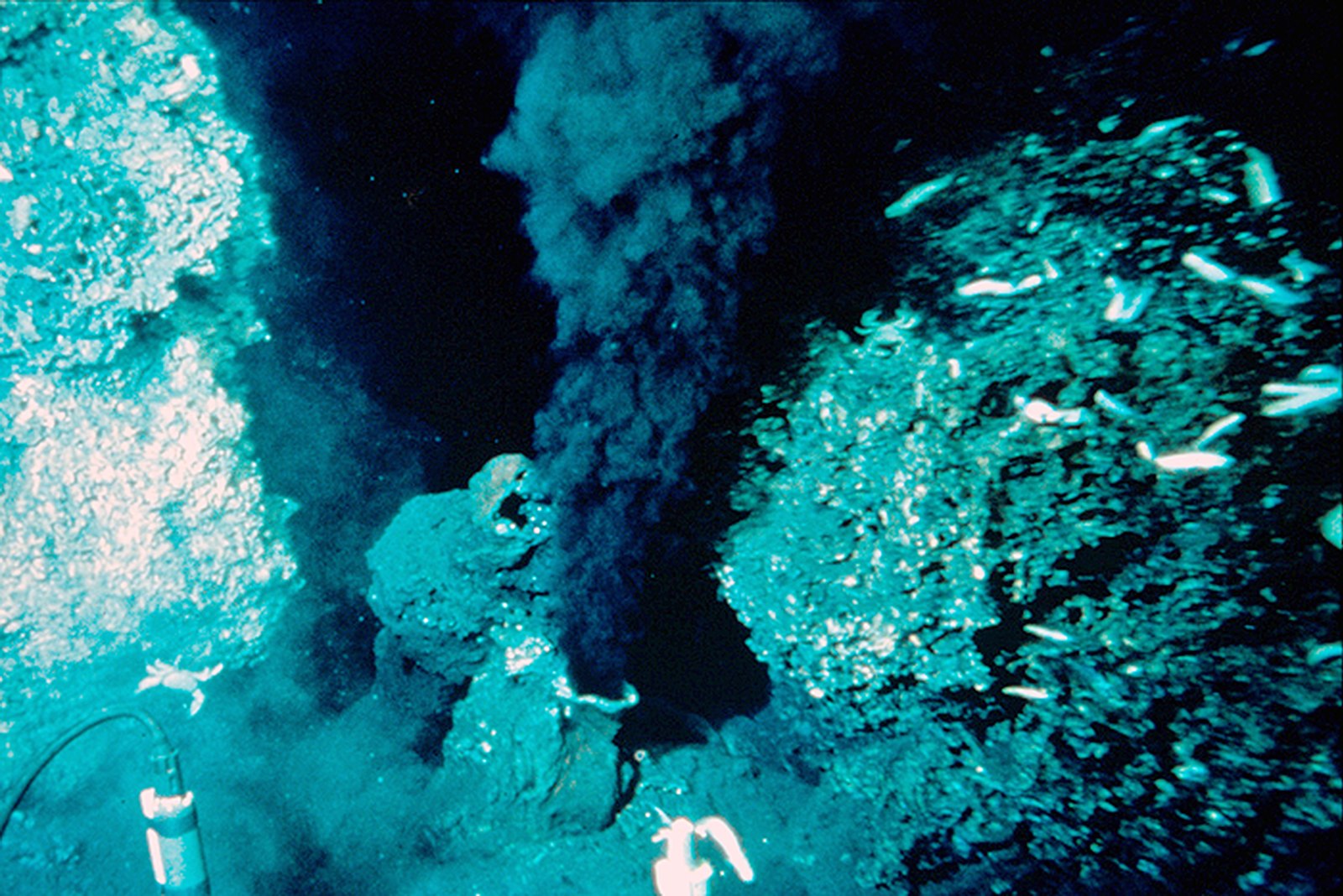
Literal gold mines are also sourced from volcanic activity, as are many other metals. Humans have had a long history of using igneous rocks and precious minerals to build industries and economies. Below, we will explore how volcanoes have transformed life on our planet, and how we have learned to harness the benefits of volcanic activity.
Hydrothermal Vents
An intriguing consequence of volcanism at Mid-Ocean Ridges are areas along the divergent boundary that heat up the seawater. This water reaches temperatures up to 380°C (716°F!). At such hot temperatures, the water can dissolve metallic elements from the igneous rocks. When that water circulates up to the rest of the ocean, it carries a surprise: the water is dark black!
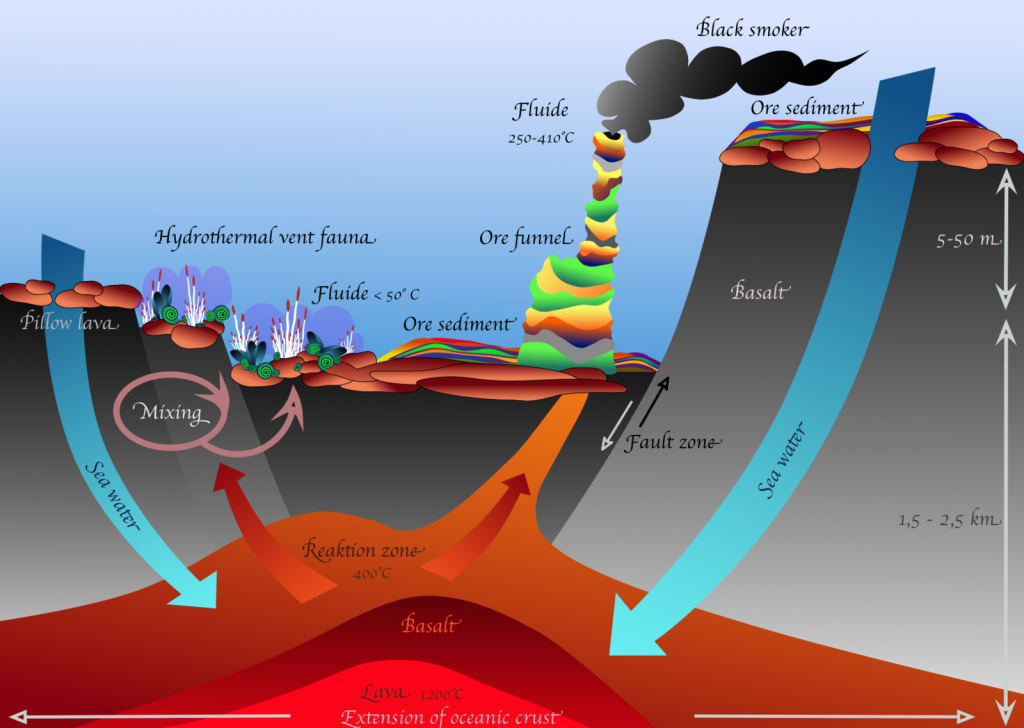
These areas of very hot, mineral-rich water are called deep-sea hydrothermal vents. They are sites for economic and precious mineral deposition, but they are also areas where diverse ecosystems can thrive deep beyond the reach of sunlight. Hydrothermal vents that belch out dark plumes of minerals along with hot water are usually called “black smokers”.
Scientists are very interested in hydrothermal vents because there is a strong indication that some of the earliest lifeforms on Earth preferred very high temperatures of over 80°C. We do not know much about the very first life on Earth, except that it had to be very similar to a single celled bacterium, and that it did not have the capability to make its energy through photosynthesis. Some scientists suggest that the first life on Earth lived close to a hydrothermal vent, where it could keep warm and make its energy through an alternative method of eating the chemicals that are released through this volcanic process.
Hot Springs and Geysers
Water and high temperature rock do not just encounter one another deep in the ocean at Mid-Ocean Ridges. This process also regularly occurs on land. Volcanic activity keeps rock beneath our surface very hot; therefore, when water meets this rock at depth it, too, becomes superheated. This very hot water will find a way to rise to the surface, often through cracks in the layers of rock, and the end result is often exciting! We will observe that hot water shoot upward from underground as a geyser.
[Video Description: A large geyser, known as Old Faithful, continuously erupts hot water and steam up around 130 feet into the air, goes back down into the ground, and then erupts more to the cheers of surrounding tourists at Yellowstone National Park.]
Perhaps the most famous geyser in the United States is Old Faithful, at Yellowstone National Park. This region is affected by hot spot volcanism, and the geyser itself predictably erupts about 17 times each day [15].
A hot spring describes hot or warm water that has quietly pooled at the surface. As you might expect from the presence of Old Faithful, there are thousands of hot springs at Yellowstone! Like hydrothermal vents, hot springs provide a wealth of chemical and heat energy for microbes to thrive, which can lead to a wide range of differently colored springs, such as the Grand Prismatic Spring in Yellowstone.
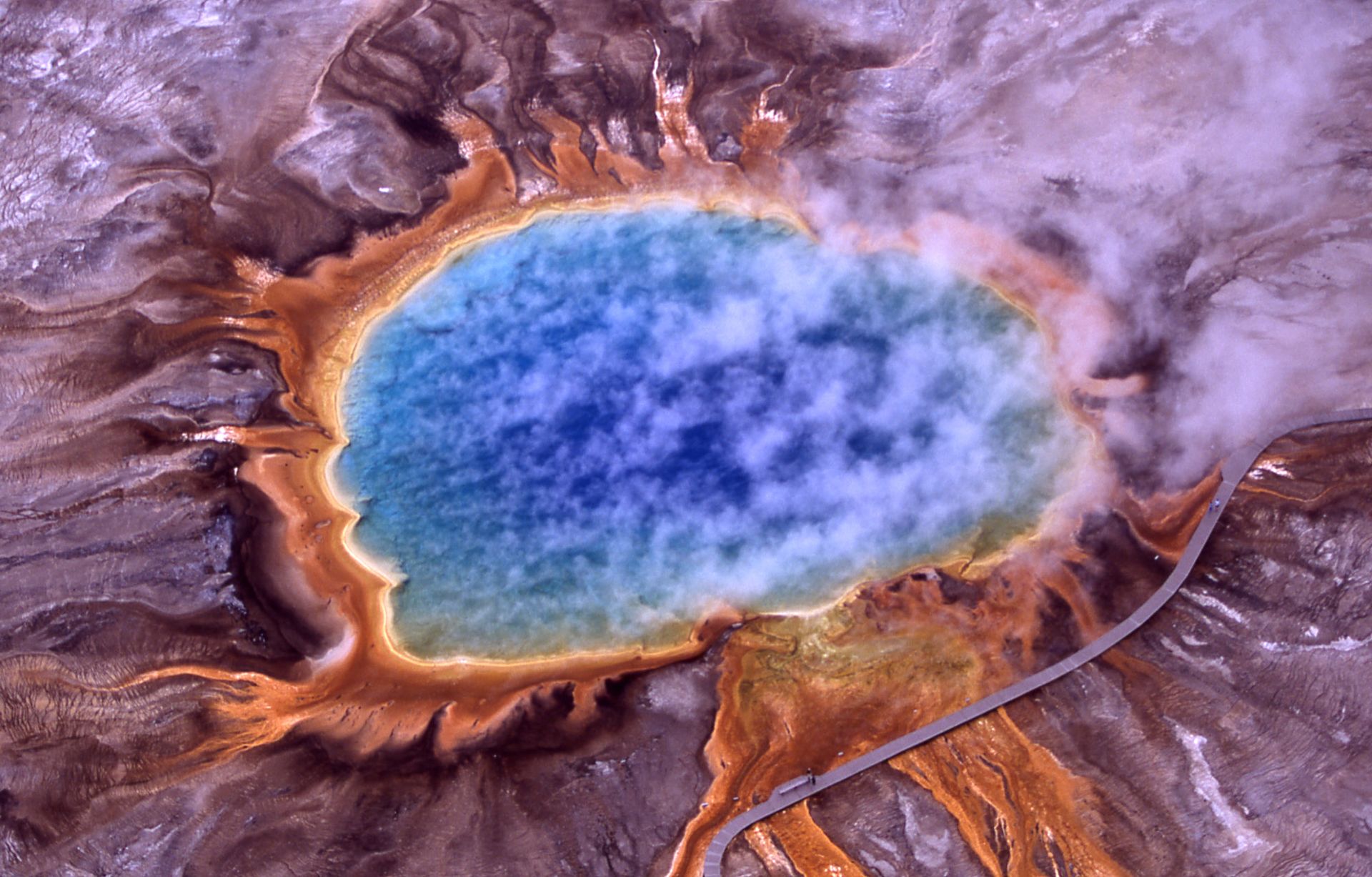
Most hot springs are known in the media for their warm water that humans (and animals!) can soak in comfortably. Japan, which sits along a subduction zone with significant volcanic activity, has built a rich culture surrounding hot springs. Some hot springs contain unique concentrations of dissolved elements and minerals that have softened skin and (reportedly) relaxed tired muscles.

Nevertheless, if you personally encounter a hot spring, DO NOT step into it, unless it has been approved as safe for human use! A lot of hot springs are extremely acidic, or extremely alkaline. Others are boiling hot, or contain toxic gases and chemicals. Nearly every year, there is a report of a tourist death or severe injury at Yellowstone National Park due to attempted bathing in the hot springs. In some of these unfortunate circumstances, the bodies of these individuals are dissolved in the acidic, boiling water within a day.
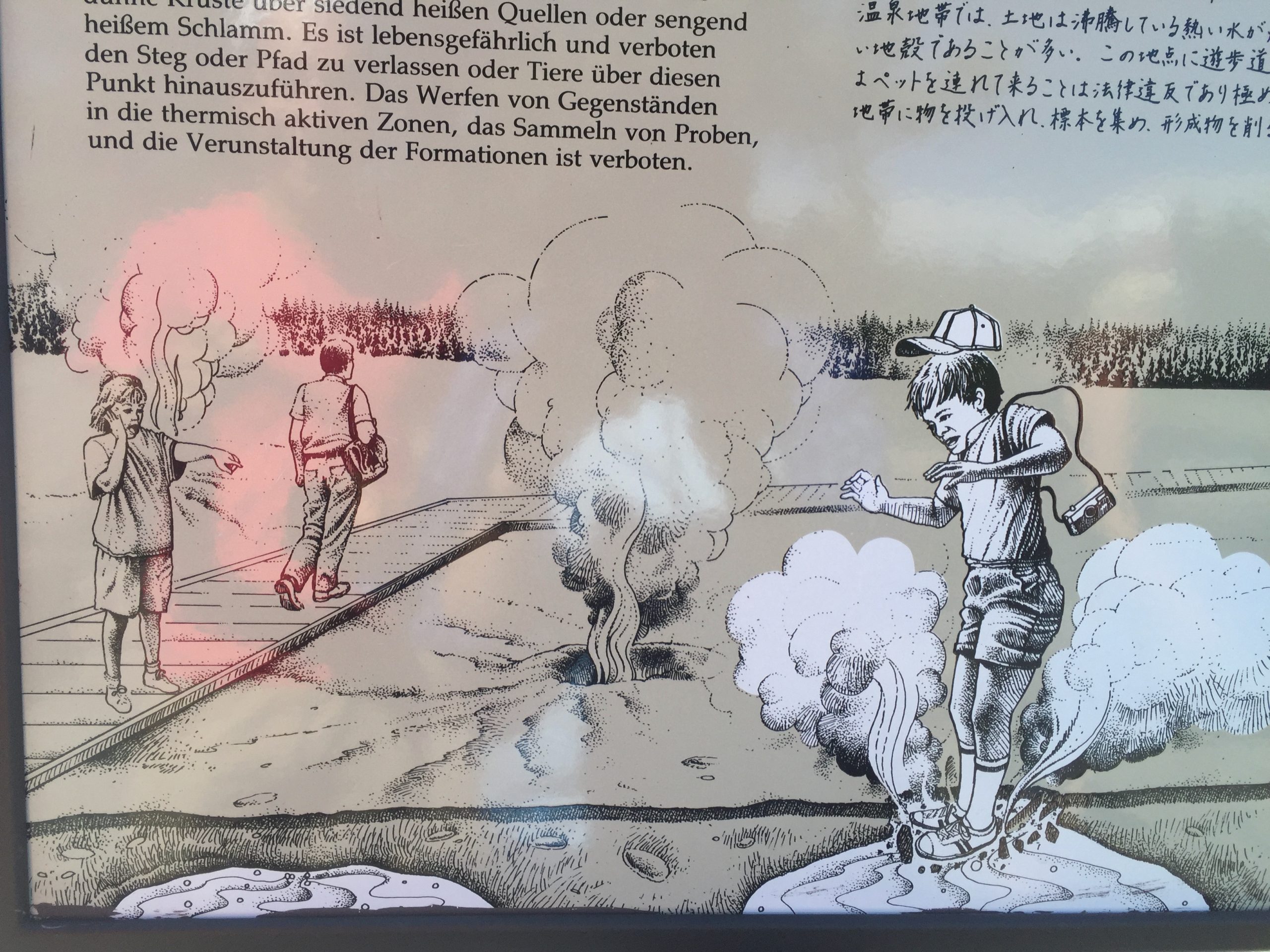
Backyard Geology: Hot Springs in Arizona! 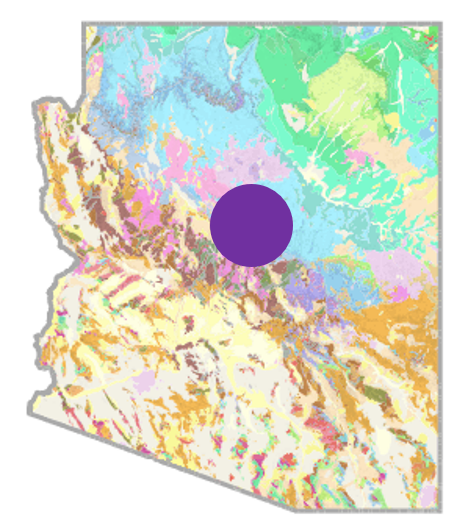
There are many hot springs throughout the United States, and Arizona is no exception! Although the majority of our volcanoes are now extinct in Northern Arizona, hot springs can also be heated by warm rock at depth, caused by the geothermal gradient. When that warm water rises, it can have the same therapeutic benefits as springs in Japan. Two popular destinations are Castle Hot Springs (60 miles away) and Verde Hot Springs (100 miles away, pictured below). The hot springs in our state were traditionally believed by the Apache and Yavapai to hold healing properties [16], and should you experience an Arizona hot spring (maybe not in the summer) it will be easy to understand why!
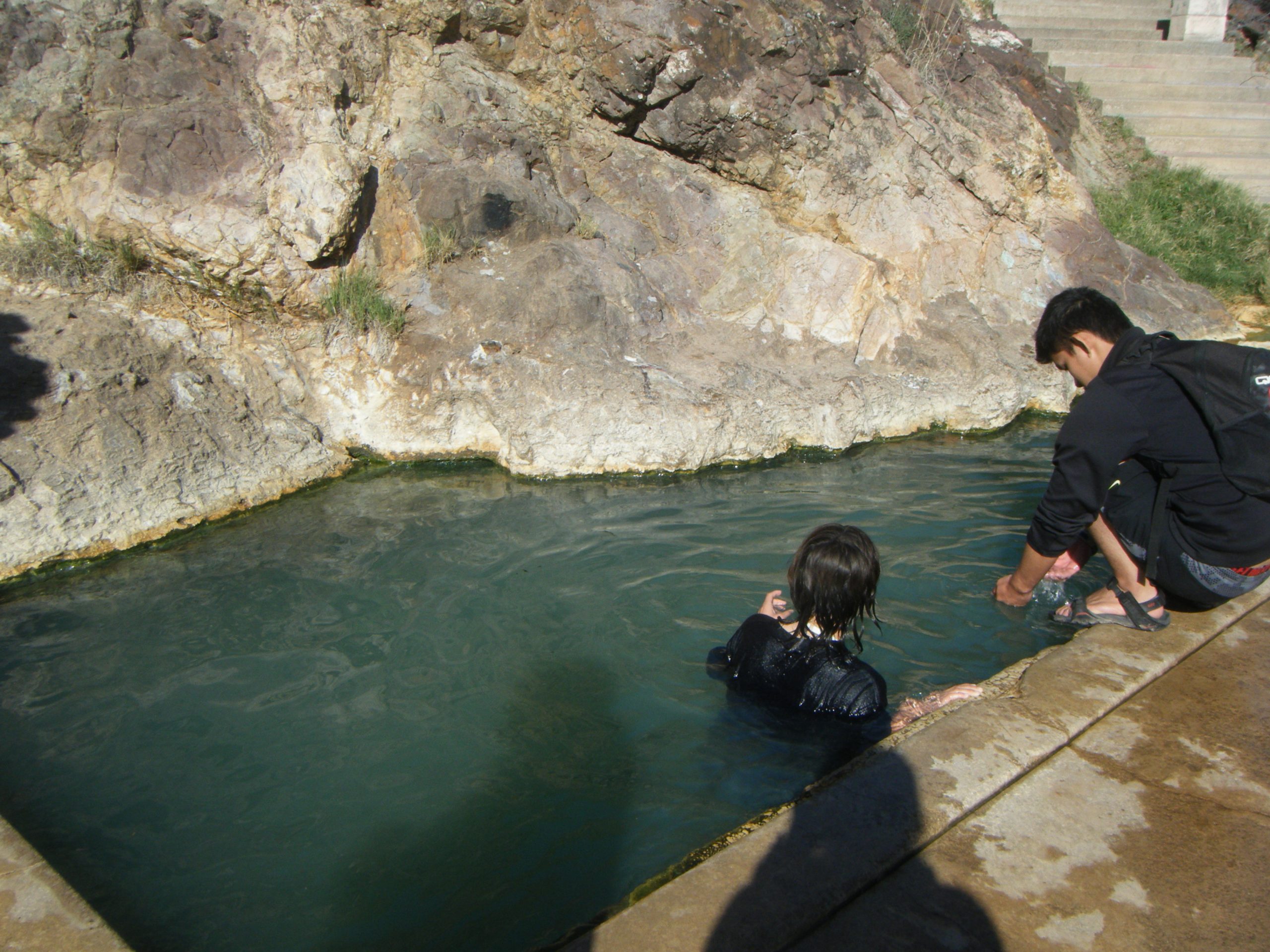
A Wealth of Resources
Most volcanic activity is great for business. Pompeii and Mount Vesuvius. The Hawaiian Islands. Arequipa and El Misti. Tokyo and Fuji-san. Historically, there have been many civilizations around volcanoes, and this was not bad luck or coincidence. When lava cools, it becomes igneous rock. When igneous rock is eventually weathered, it provides the land with fertile soil for agriculture.
Volcanoes also leave deposits of precious minerals such as gold, silver, copper, lead, zinc, iron, and nickel. Many of these are precipitated by the “black-smoker” hydrothermal vents. Volcanoes more commonly deposit igneous rocks such as granite that have been used as sturdy building materials.
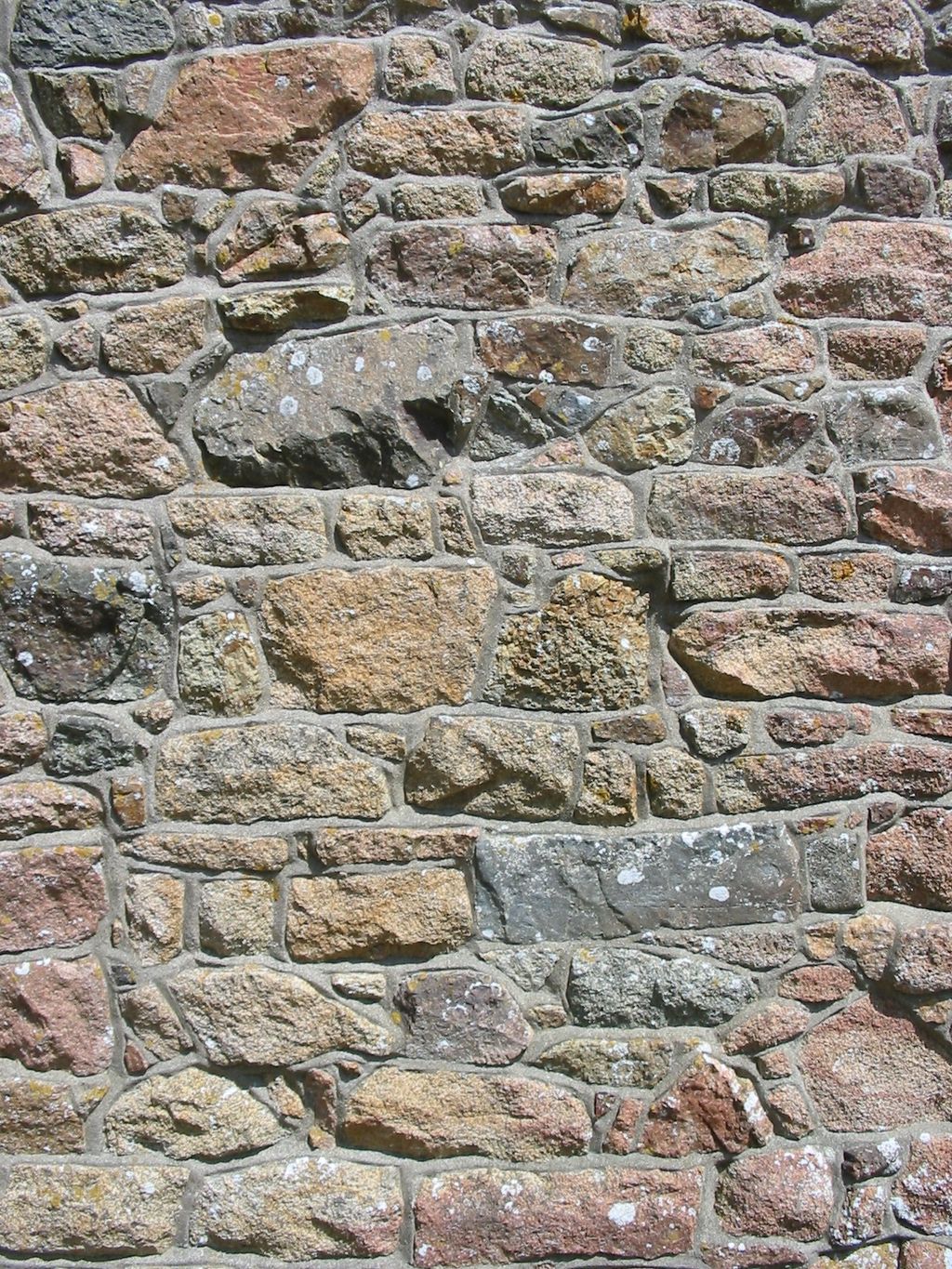
Some of the most amazing landscapes are near volcanoes because volcanic activity builds land and creates breathtaking scenery. The volcanoes themselves are economically vital for many regions because of the recreational activity and tourism they bring [1]. As terrifying as an eruption at Yellowstone may be, this region is one of the most frequented parks by international tourists in the United States!
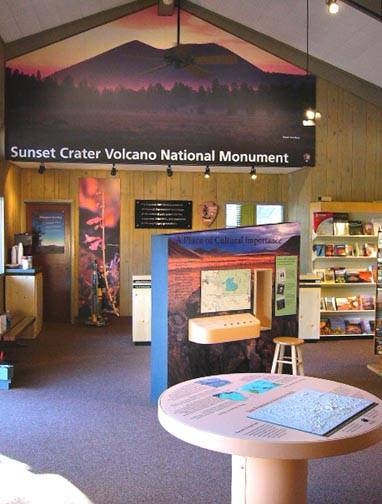
Geothermal Energy

Geothermal energy is becoming a rising alternative source of energy. “Geothermal” references the fact that heat is being sources from the Earth, or in this situation, volcanic activity at depth. Volcanic activity generates heat, which can be harnessed to create electricity [1]. In some regions, there is enough geothermal power to completely replace our more traditional methods of generating electricity such as burning fossil fuels, and this method does not produce additional greenhouse gases.
Which regions would benefit most from geothermal energy? Follow the volcanoes. Hawaii, particularly at the world’s most active volcano of Kilauea, is an excellent source of American geothermal energy. Iceland also significantly benefits from this natural resource since it sits along the Mid-Atlantic Ridge. Around 85% of the homes in that country are already powered by geothermal energy [17].

***See 5.8 for Text and Media Attributions
molten rock that has erupted at the Earth's surface due to volcanic processes.
A region along Earth's lithosphere where at least two tectonic plates move apart from one another.
volcanically ejected rock fragments or particles that are less than 2 mm.
It’s important to classify slope failures so that we can understand what causes them and learn how to mitigate their effects. The three criteria used to describe slope failures are:
- Material: Type of material that failed (typically either bedrock or unconsolidated sediment)
- Motion: How the material moved (fall, slide, or flow).
- Rate: Speed at which the material moved.
Three types of motion associated with slope failure are:
- Fall: Material drops through the air, vertically or nearly vertically.
- Slide: Material moves as a cohesive mass along a sloping surface.
- Flow: Material moves like a fluid.
Unfortunately it’s not normally that simple. Many slope failures involve two of these types of motion, some involve all three, and in many cases, it’s not easy to tell how the material moved. The types of slope failure that we’ll cover here are summarized in Table 11.1, though there are other types of mass wasting.
[Skip Table] | |||
Failure Type | Type of Material | Type of Motion | Rate of Motion |
---|---|---|---|
Rock fall | Bedrock | Fall | Extremely rapid |
Rock slide | Bedrock | Slide | Very slow to extremely rapid |
Rock avalanche | Bedrock (slides then breaks into smaller fragments) | Flow | Extremely rapid |
Creep or solifluction | Unconsolidated materials (rock fragments, soils) | Flow or slide | Extremely slow |
Slump | Unconsolidated sediments | Slide | Very slow to moderate |
Mudflow | Unconsolidated sediments (very small silt and clay) | Flow | Moderate to Extremely rapid |
Debris flow | Unconsolidated sediments (Sand, gravel, and larger fragments) | Flow | Rapid to Extremely rapid |
Rock Fall
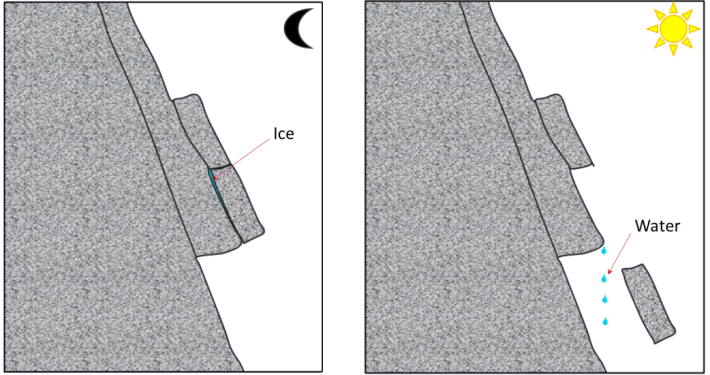
Rock fragments can break off relatively easily from steep bedrock slopes, most commonly due to frost-wedging in areas where there are many freeze-thaw cycles per year. When water freezes to form ice, its volume increases by about 8%, this causes fractures to enlarge. When the ice melts, more water can fill the larger crack, and refreeze. This process over time can cause large pieces of solid rock to fall (Fig 11.2.1). However, it can occur due to other triggers, particularly heavy rain, which adds weight or loosens material.
A rock fall in Verde Valley, Arizona, caused tons of solid material to fall to the valley below, destroying an RV but stopping short of hitting the house (Figure 11.2.2). Luckily no one was hurt in this rock fall, but living at the base of a cliff can be very dangerous and a fall is likely to happen again.

Rock Slide
A rock slide is the sliding motion of rock along a sloping surface. In most cases, the movement is parallel to a fracture, bedding, or metamorphic foliation plane, and it can range from very slow to moderately fast.
On June 23, 1925, a 38 million cubic meter rock slide occurred next to the Gros Ventre River (pronounced “grow vont”) near Jackson Hole, Wyoming. Large boulders dammed the Gros Ventre River and ran up the opposite side of the valley several hundred vertical feet. The dammed rivercreated Slide Lake, and two years later in 1927, lake levels rose high enough to destabilize the dam. The dam failed and caused a catastrophic flood that killed six people in the small downstream community of Kelly, Wyoming.

A combination of three factors caused the rock slide: 1) heavy rains and rapidly melting snow saturated the sandstone causing the underlying shale to lose its shear strength, 2) the Gros Ventre River cut through the sandstone creating an <span class="glossaryLink" style="border-bottom: 1px dotted #000000 !important;text-decoration: none !important;color: #000000 !important" aria-describedby="tt" data-cmtooltip="
">oversteepened slope, and 3) soil on top of the mountain became saturated with water due to poor drainage. The cross-section diagram shows how the parallel bedding planes between the sandstone and clay/limestone offered little friction against the slope surface as the river undercut the sandstone. Lastly, the rockslide may have been triggered by an earthquake. (3)
Rock Avalanche
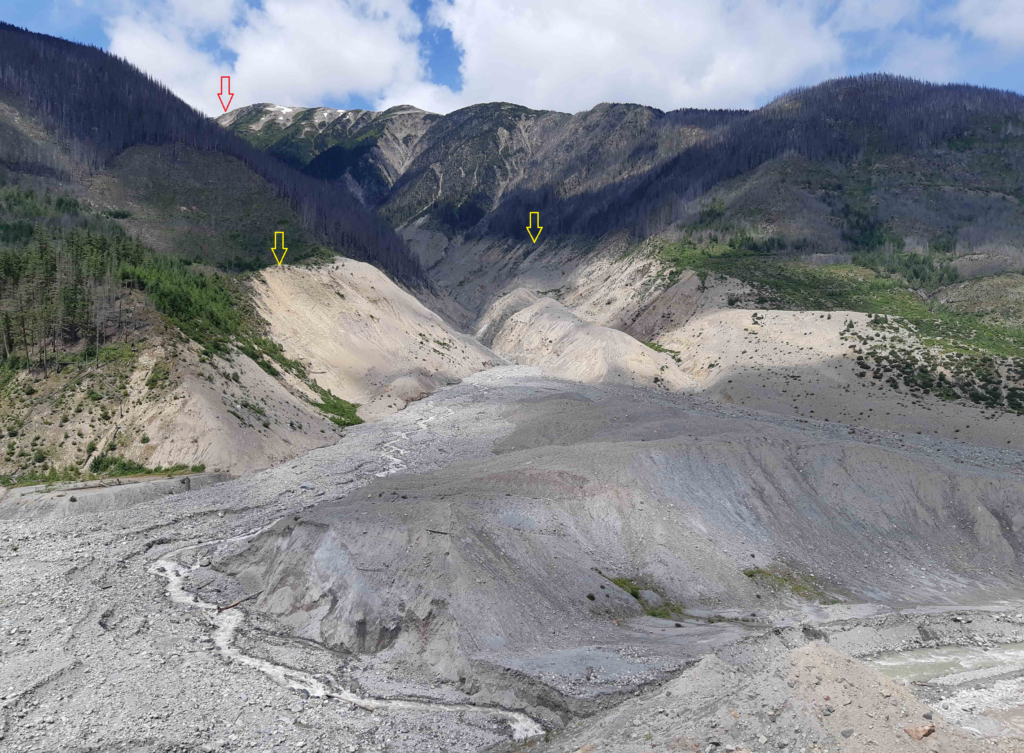
If a rock slides and then starts moving quickly (meters per second), the rock is likely to break into many small pieces, and at that point it turns into a rock avalanche in which the large and small fragments of rock move in a fluid manner supported by a cushion of air within and beneath the moving mass. The 2010 slide at Mount Meager (north of Vancouver, British Columbia, Canada) was a rock avalanche, and is the largest slope failure in Canada during historical times (Figure 11.2.5). Though no one was harmed during this event, there is a great potential for damage and loss of life from this type of mass wasting. (1)
Creep or Solifluction
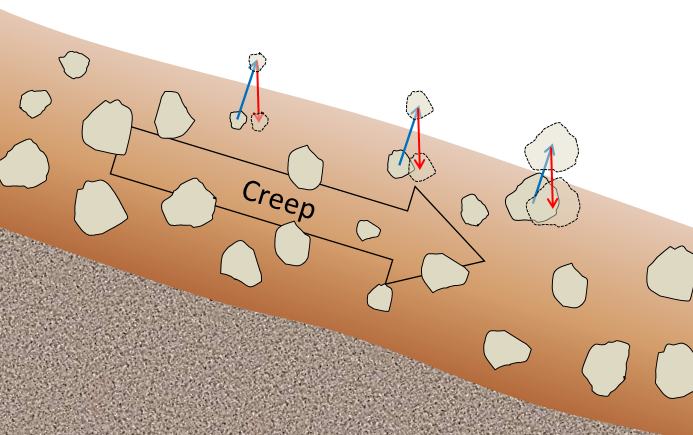
The very slow—millimeters per year to centimeters per year—movement of soil or other unconsolidated material on a slope is known as creep. Creep, which normally only affects the upper several centimeters of loose material, is typically a type of very slow flow, but in some cases, sliding may take place. Creep can be facilitated by freezing and thawing because, as shown in Figure 11.2.6, particles are lifted perpendicular to the surface by the growth of ice crystals within the soil, and then let down vertically by gravity when the ice melts. The same effect can be produced by frequent wetting and drying of the soil. In cold environments, solifluction is a more intense form of freeze-thaw-triggered creep. Creep is most noticeable on moderate-to-steep slopes where trees, fence posts, or grave markers are consistently leaning in a downhill direction. In the case of trees, they try to correct their lean by growing upright, and this leads to a curved lower trunk, shown on Figure 11.2.7.
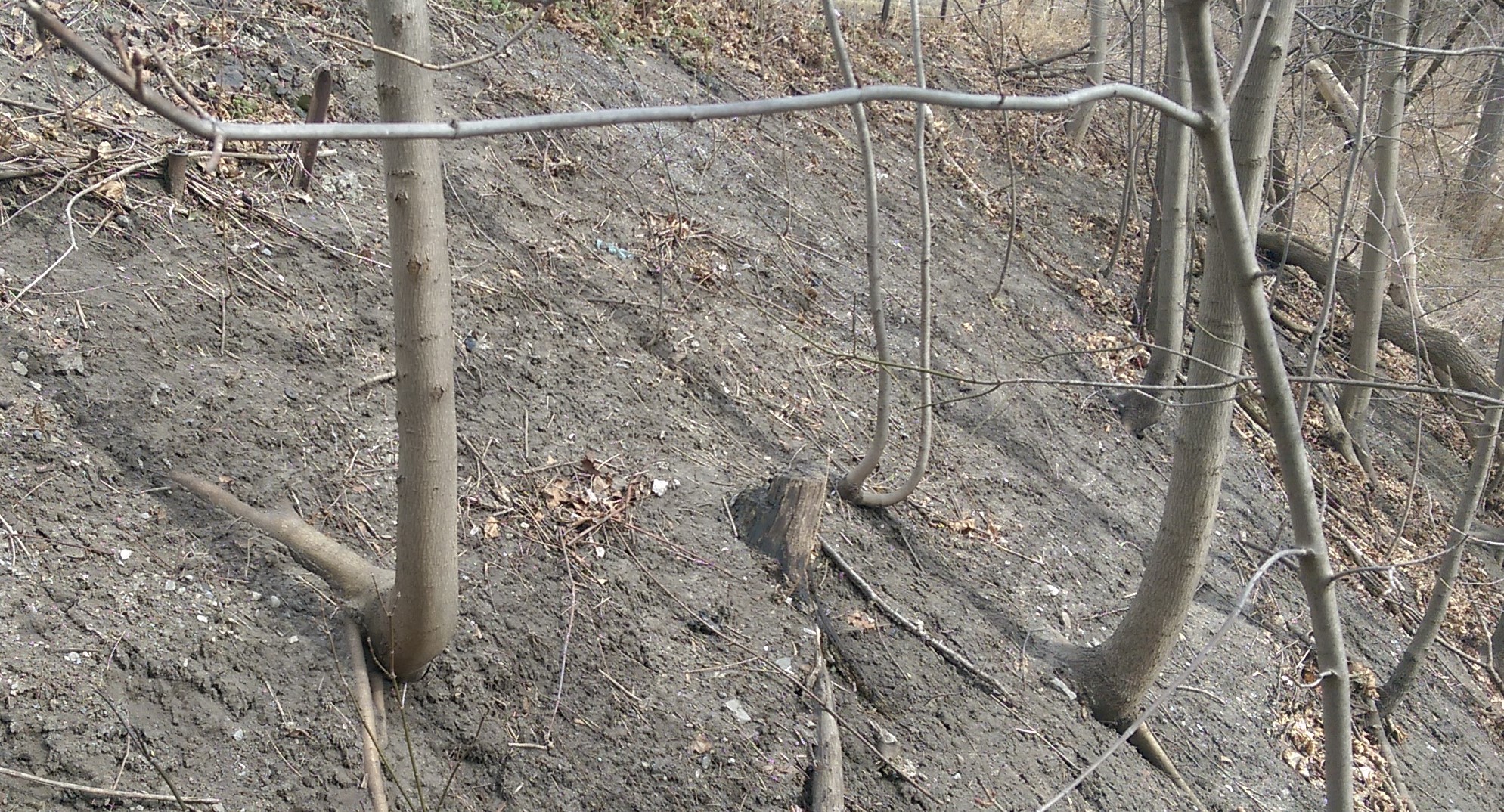
Slump
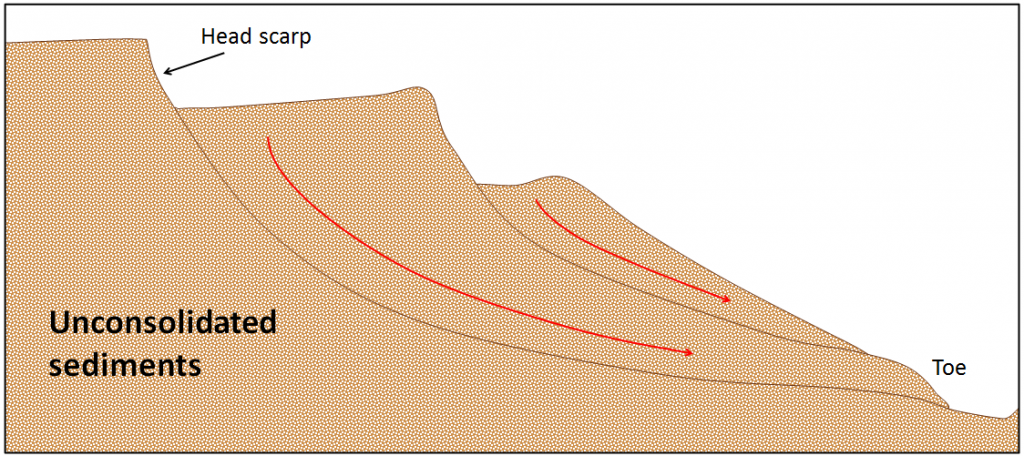
Slump is a type of slide (movement as a mass) that takes place within thick unconsolidated deposits (typically thicker than 10 meters). Slumps involve movement along one or more curved failure surfaces, with downward motion near the top and outward motion toward the bottom (Figure 11.2.8). They are typically caused by an excess of water within these materials on a steep slope.
An example of a slump in the Lethbridge area of Alberta is shown in Figure 11.2.9. This feature has likely been active for many decades, and moves a little more whenever there are heavy spring rains and significant snowmelt runoff. The toe of the slump is failing because it has been eroded by the small stream at the bottom.
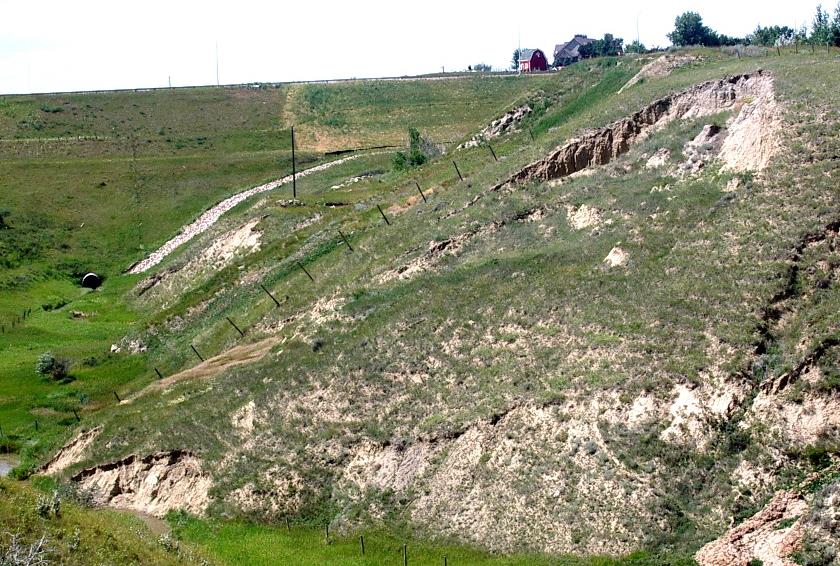
Mudflows and Debris Flows
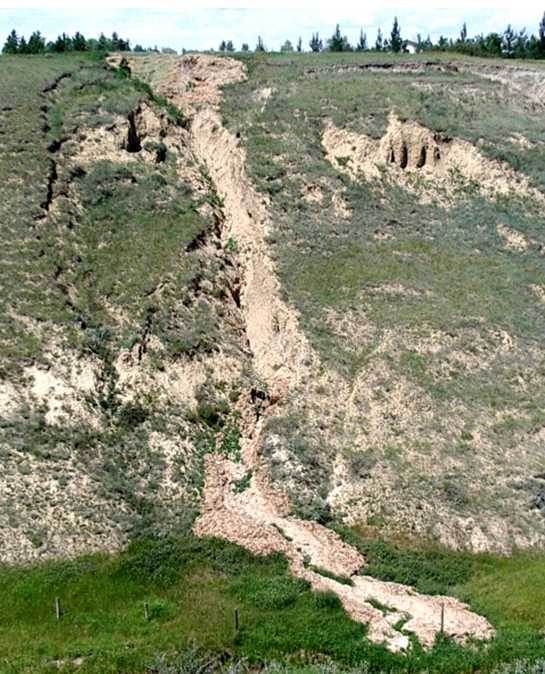
As you saw previously, when a mass of sediment becomes completely saturated with water, the mass loses strength, to the extent that the grains are pushed apart, and it will flow, even on a gentle slope. This can happen during rapid spring snowmelt or heavy rains, and is also relatively common during volcanic eruptions because of the rapid melting of snow and ice. (A mudflow or debris flow on a volcano or during a volcanic eruption is a lahar.) If the material involved is primarily sand-sized or smaller, it is known as a mudflow, such as the one shown in Figure 11.2.10.

If the material involved is gravel sized or larger, it is known as a debris flow. Because it takes more gravitational energy to move larger particles, a debris flow typically forms in an area with steeper slopes and more water than does a mudflow. In many cases, a debris flow takes place within a steep stream channel, and is triggered by the collapse of bank material into the stream. This creates a temporary dam, and then a major flow of water and debris when the dam breaks. Large amounts of debris can be carried.
We may receive all the heat we need on the Earth's surface from sunlight, but if you dig a hole just a few feet deep, you might notice that the surrounding soil is much cooler. This happens because much of the sediment is insulated from the sun's energy. Nevertheless, if you had the ability to dig miles and miles into the crust, you would find that the temperature of the rock would start to significantly increase!

This increase in temperature with depth in the Earth's crust is called the geothermal gradient. In general, we can expect temperatures to increase about 25°C for each kilometer of depth. This sounds like a significant increase in heat, but once we pass the 100 km mark, temperatures really take off!
As we learned in the second chapter, the Earth's iron core is wickedly hot! Such heat powers plate tectonics, and when combined with other factors, it can lead to partial melting in the upper mantle. This melted, molten rock occasionally rises to the Earth's surface and when it does, watch out!
A volcano will form above a chamber or plume of magma. There is a wide variety of volcanoes, and some are more explosive than others. Molten lava is far from the only hazard; volcanic eruptions can cause massive damage with ashfall, pyroclastic flows, toxic gases, and deadly landslides. There are even a few volcanoes considered so dangerous that they are termed "supervolcanoes", and they have the potential to drastically impact society for generations.
Although volcanoes are hazardous, they are a continuous boon for the global economy. The precious minerals and useful rocks formed by volcanic processes have benefited civilization for thousands of years. In the modern economy, we use volcanism as a source of alternative energy and igneous rocks in trades ranging from construction to cosmetics.
Learning Objectives
- Describe the size, shape, and eruption style of volcanoes and how they form/
- Identify the major volcanic hazards and their effects on humans and property.
- Explain how scientists monitor volcanoes and where volcanoes can be found.
- Explain how volcanic processes benefit society.
Chapters
Image by USGS (Public Domain).
earthquakes that precede larger earthquakes in the same location
Fine particles, liquid droplets, or gases that are ejected into the atmosphere by man-made pollution or volcanic eruptions. Aerosols have the ability to reflect sunlight back toward space and cause the planet to experience colder temperatures.
Geology is an umbrella science that encompasses all biological, chemical, and physical processes that act on the planet and make up our world. In the same way that the human body can be divided into systems (circulatory, digestive, endocrine, etc.), the Earth can be described as a complex entity that encompasses many different systems all acting on one another. There are 5 main systems, or spheres, found on Earth: geosphere, biosphere, hydrosphere, atmosphere, and cryosphere.
Geosphere ("geo" = Earth)
The geosphere encompasses all physical material that makes up the interior and surface of the Earth. This includes all rocks and minerals, but is also includes the active processes that link these rocks and minerals together. While many tend to focus on the three types of rocks: igneous, sedimentary, and metamorphic, the forces of plate tectonics and weathering actively change rocks from one type to another. This system is referred to as the Rock Cycle. This is the focus of most physical geology courses and will be the main framework for this text. Because the geosphere describes the physical structure of the Earth, it is integral to all other spheres as well.
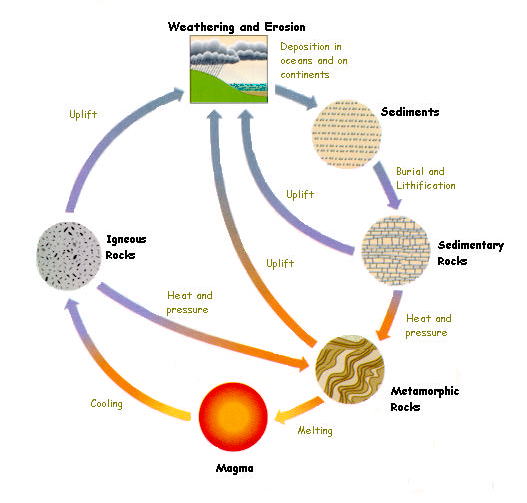
Biosphere ("bio" = life)
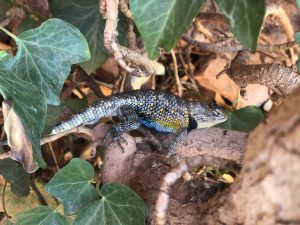
The biosphere is made up of all living things, as well as the ecosystems that support that life. Obviously, life can be found in many different places, from the tops of mountains to the bottoms of oceans, in wet and dry places, in cold and hot environments. The biosphere therefore overlaps and interacts with all other spheres.
Hydrosphere ("hydro" = water)
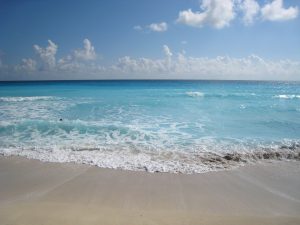
The Earth has been described as the “Blue Marble” in space due to the presence of enormous amounts of water on its surface. All of the liquid water found on earth makes up the hydrosphere. As water flows on the Earth’s surface, it changes landscapes and nourishes life, and interacts with all other spheres.
Atmosphere ("atmo" = vapor)
All of the gases that circulate around and surround the earth are a part of the atmosphere. This includes the air we breathe, the gases that are responsible for global weather and climate, as well as the ones that burn up meteorites as they plummet toward the Earth’s surface. The atmosphere is further broken into layers: troposphere, stratosphere, mesosphere, thermosphere, and exosphere.
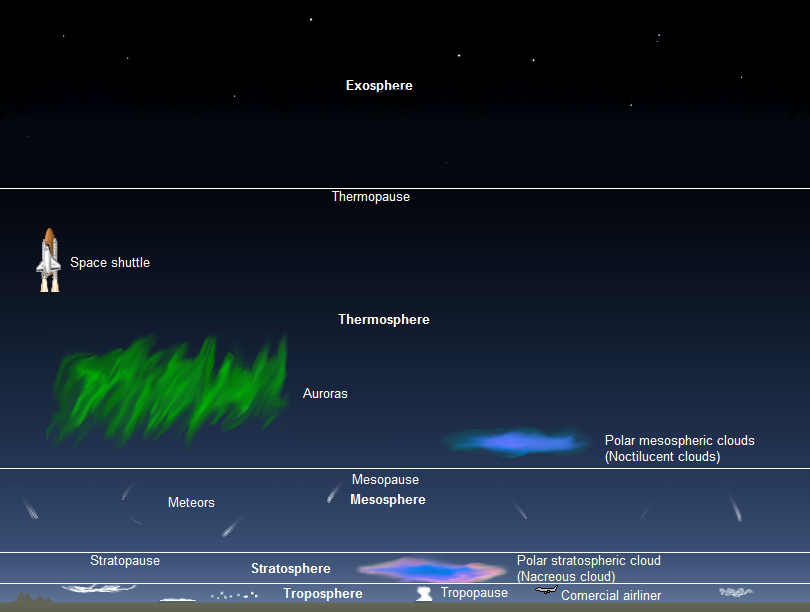
Cryosphere ("cryo" = frozen)
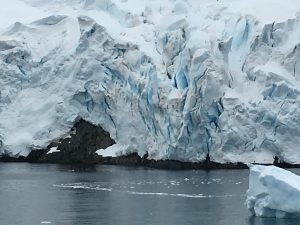
Cryo- comes from a Greek word meaning "frost." The cryosphere encompasses all of frozen water on the Earth. Typically found at high elevations and at the poles, ice can have dramatic impacts on landscapes (geosphere), provide sources of liquid water (hydrosphere), and support ecosystems for living things (biosphere).
Integrated systems
While it is easier to look at each system independently, they are all integrated on the Earth. Each one is constantly acting and interacting with another on our dynamic planet.
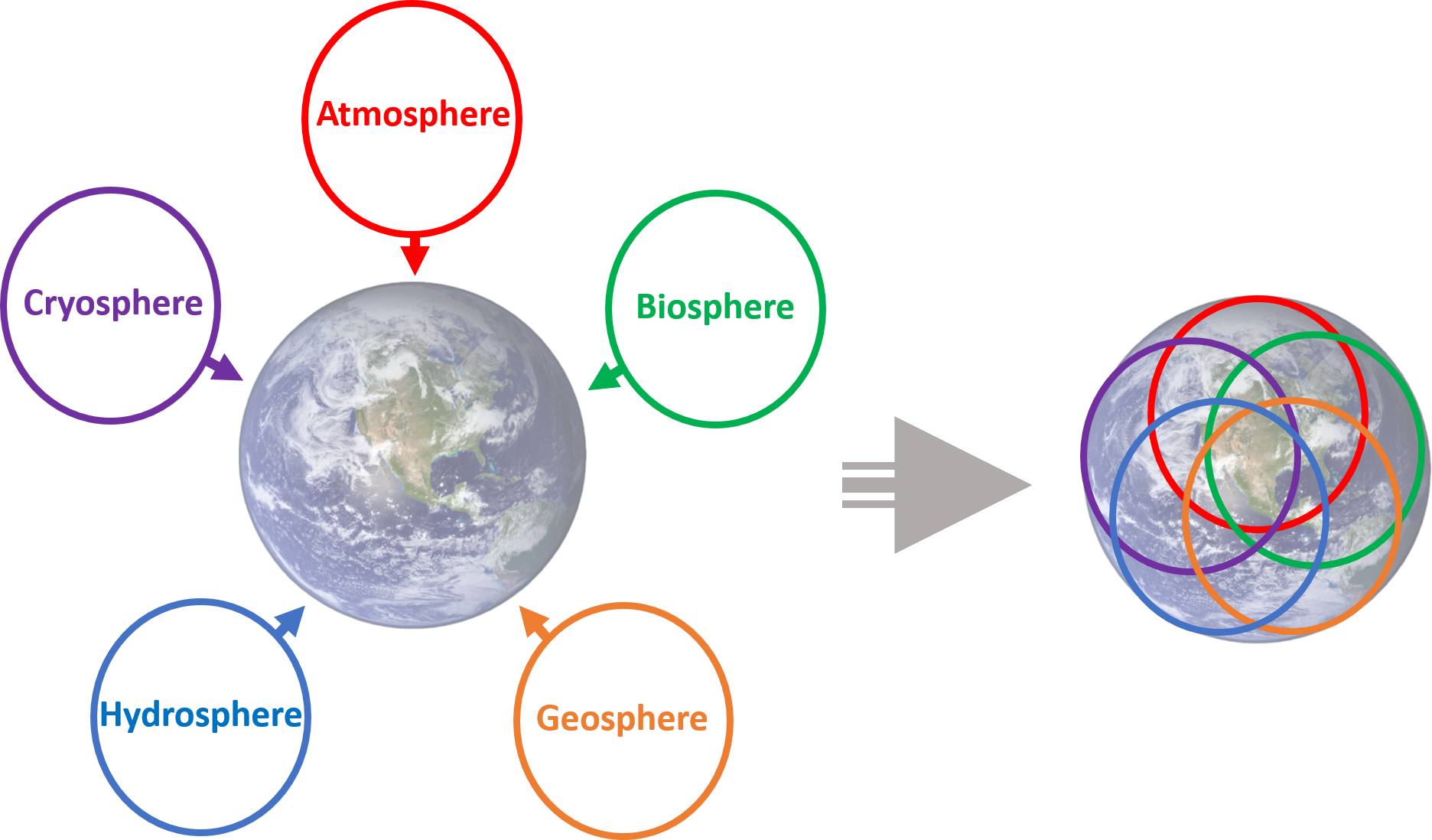
H2O, or the water molecule, is constantly changing. It is the only substance on Earth that is present in all states of matter - solid, liquid, and gas. The cycling of water throughout the Earth, also called the hydrologic cycle, describes how water moves through various environments on Earth. The largest reservoir of water is in the ocean, and it exists there as a liquid. Through the process of evaporation, it becomes a gas. At that point, it may crystallize as a solid (snow or ice) and deposit on land. It could then melt, forming a liquid again, and run off the landforms, eternally shaping the Earth. It can be taken up into plants, aiding in photosynthesis, and released as a gas through the process of transpiration. The possibilities of pathways for water to travel on Earth are endless.
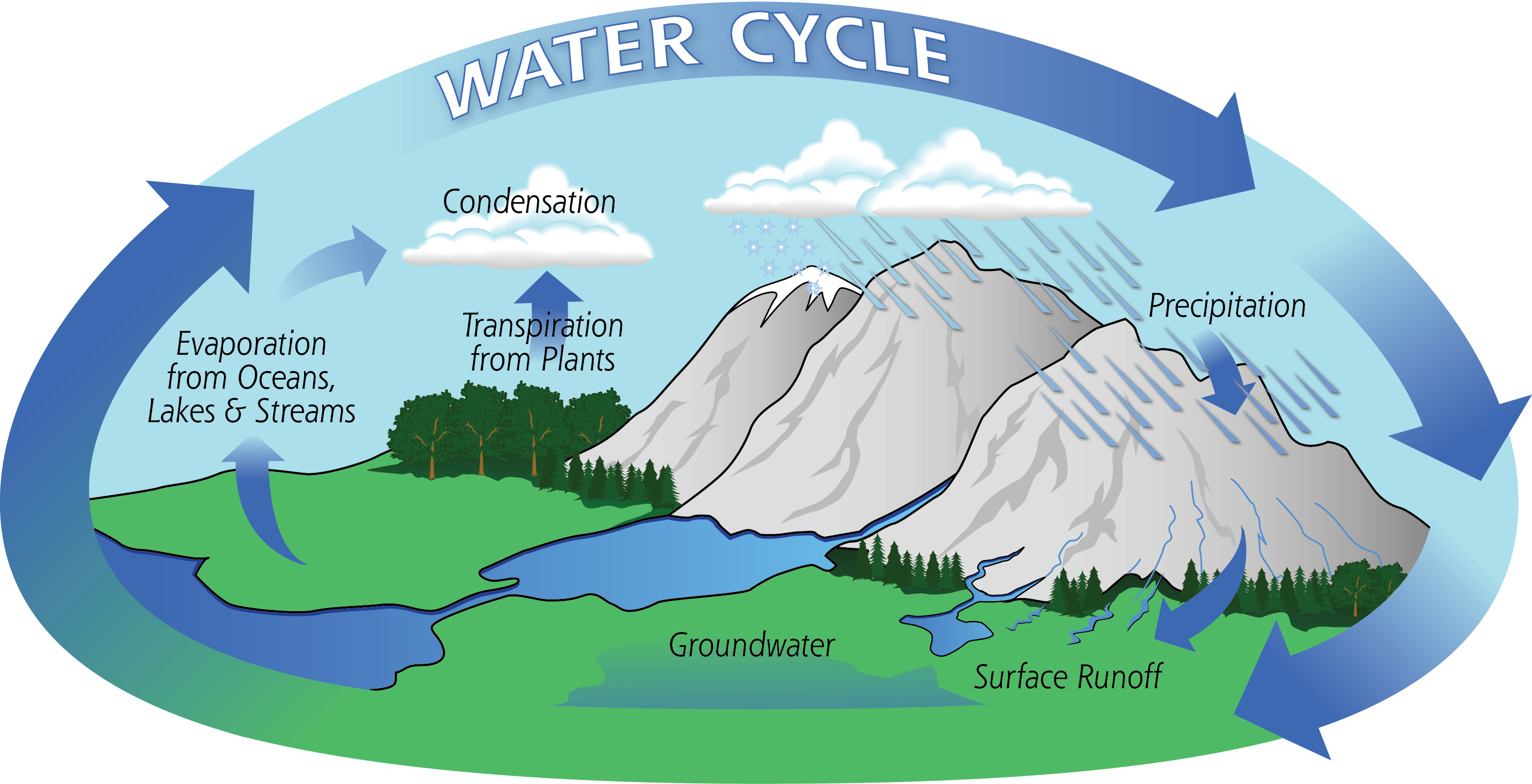
Backyard Geology: Spheres intersect for one of the 7 Natural Wonders of the World
Arizona is known as the "Grand Canyon State" because of the dramatic landscape that unfolds at the intersection of brightly colored flat-lying sedimentary rocks and a dynamic river system. This landscape evolved over millions of years while the underlying rocks were uplifted by Plate Tectonics, and the Colorado River cut down through soft sedimentary rocks..
***See 1.5 for Text and Media Attributions
The world's greatest Mass Extinction event that occurred around 252 million years ago and resulted in the loss of 95% of ocean life and 70% of life on land.
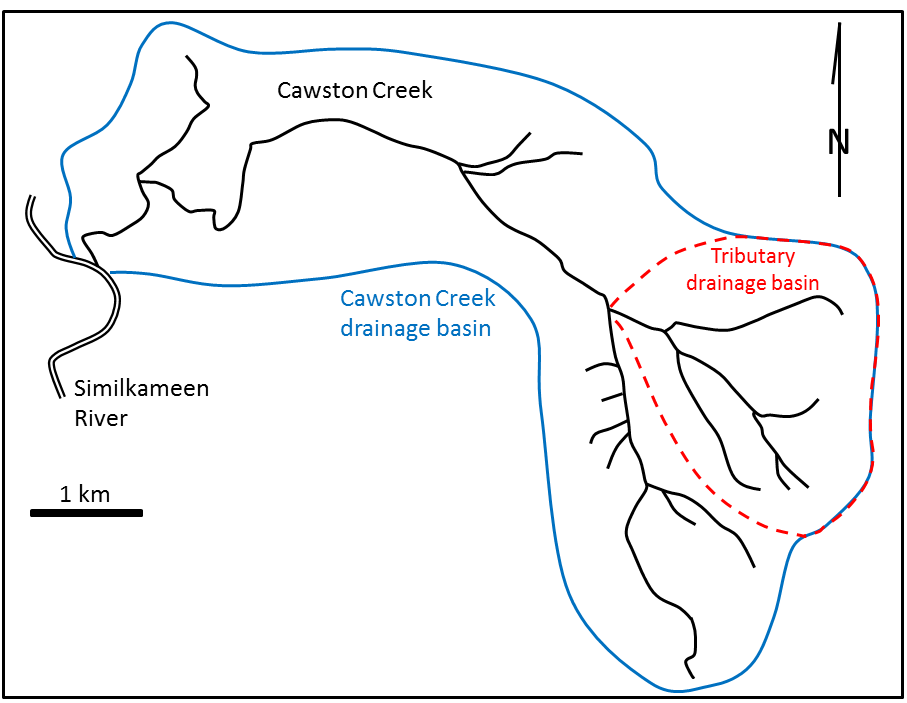
A stream is a body of flowing surface water of any size, ranging from a tiny trickle to a mighty river. The area from which the water flows to form a stream is known as its drainage basin. All of the precipitation (rain or snow) that falls within a drainage basin eventually flows into its stream, unless some of that water is able to cross into an adjacent drainage basin via groundwater flow. An example of a drainage basin is shown in Figure 13.2.1.
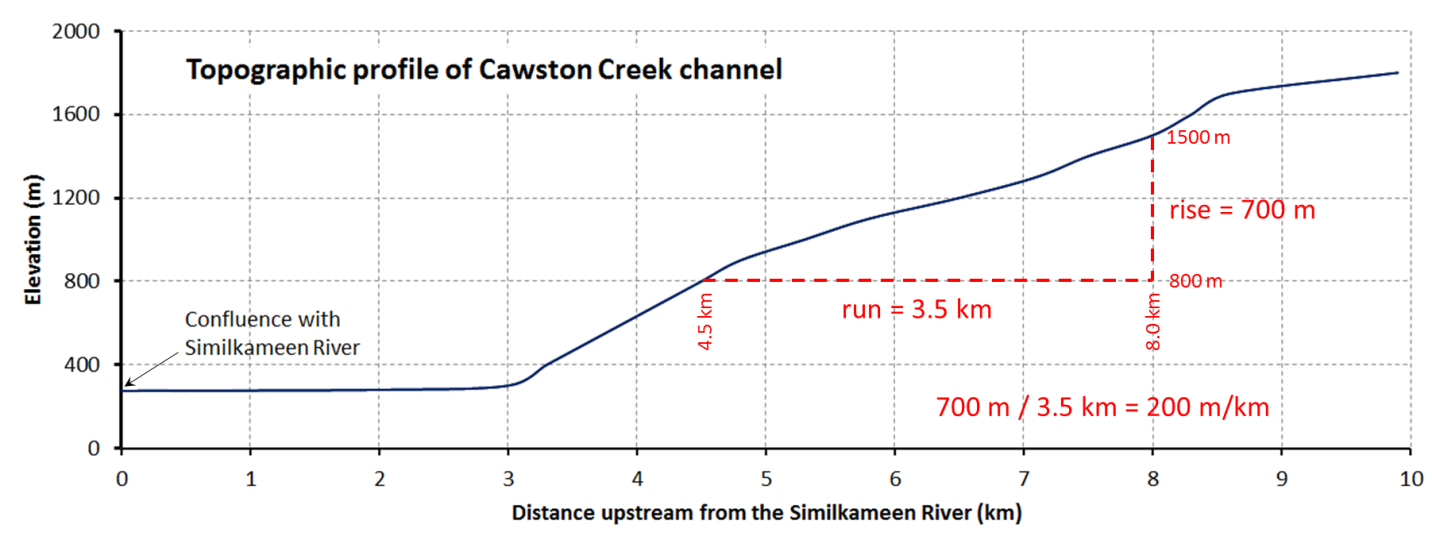
Cawston Creek is a typical small drainage basin (approximately 25 square kilometres) within a very steep glaciated valley. As shown in Figure 13.2.2, the upper and middle parts of the creek have steep gradients (averaging about 200 metres per kilometre but ranging from 100 to 350 metres per kilometre), and the lower part, within the valley of the Similkameen River, is relatively flat (less than 5 metres per kilometre). The shape of the valley has been controlled first by tectonic uplift (related to plate convergence), then by pre-glacial stream erosion and mass wasting, then by several episodes of glacial erosion, and finally by post-glacial stream erosion. The lowest elevation of Cawston Creek (275 metres at the Similkameen River) is its base level. Cawston Creek cannot erode below that level unless the Similkameen River erodes deeper into its flood plain (the area that is inundated during a flood).
Metro Vancouver’s water supply comes from three large drainage basins on the north shore of Burrard Inlet, as shown in Figure 13.2.3. This map illustrates the concept of a drainage basin divide. The boundary between two drainage basins is the height of land between them. A drop of water falling on the boundary between the Capilano and Seymour drainage basins (a.k.a., watersheds), for example, could flow into either one of them.

The pattern of tributaries within a drainage basin depends largely on the type of rock beneath, and on structures within that rock (folds, fractures, faults, etc.). The three main types of drainage patterns are illustrated in Figure 13.2.4. Dendritic patterns, which are by far the most common, develop in areas where the rock (or unconsolidated material) beneath the stream has no particular fabric or structure and can be eroded equally easily in all directions. Examples would be granite, gneiss, volcanic rock, and sedimentary rock that has not been folded. Most areas of British Columbia have dendritic patterns, as do most areas of the prairies and the Canadian Shield. Trellis drainage patterns typically develop where sedimentary rocks have been folded or tilted and then eroded to varying degrees depending on their strength. The Rocky Mountains of B.C. and Alberta are a good example of this, and many of the drainage systems within the Rockies have trellis patterns. Rectangular patterns develop in areas that have very little topography and a system of bedding planes, fractures, or faults that form a rectangular network. Rectangular drainage patterns are rare in Canada.
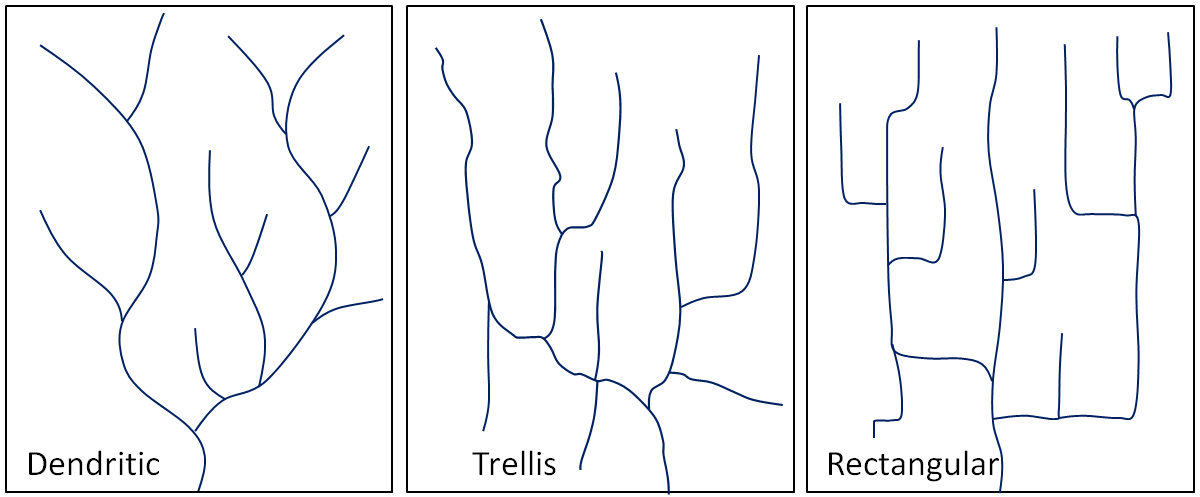
In many parts of Canada, especially relatively flat areas with thick glacial sediments, and throughout much of Canadian Shield in eastern and central Canada, drainage patterns are chaotic, or what is known as deranged (Figure 13.2.5, left). Lakes and wetlands are common in this type of environment.
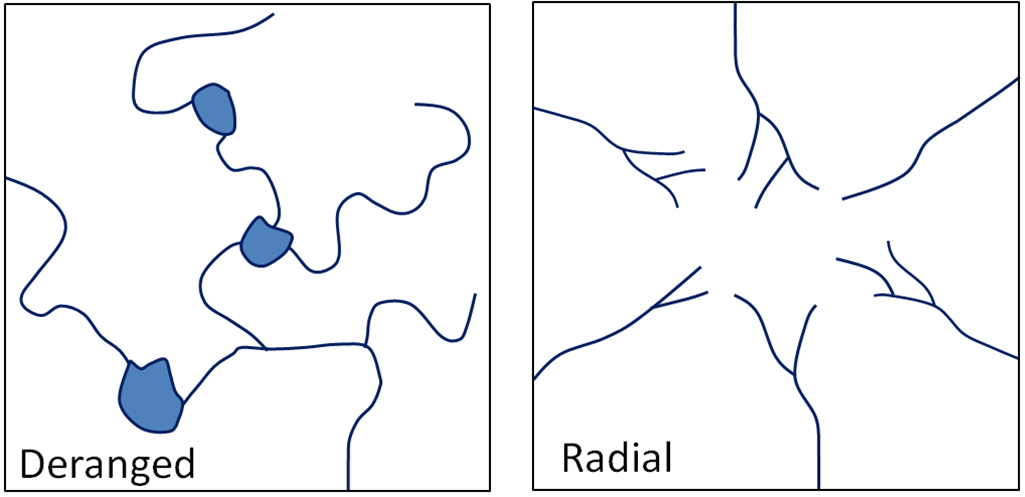
A fourth type of drainage pattern, which is not specific to a drainage basin, is known as radial (Figure 13.2.5, right). Radial patterns form around isolated mountains (such as volcanoes) or hills, and the individual streams typically have dendritic drainage patterns.
Over geological time, a stream will erode its drainage basin into a smooth profile similar to that shown in Figure 13.2.6. If we compare this with an ungraded stream like Cawston Creek (Figure 13.2.2), we can see that graded streams are steepest in their headwaters and their gradient gradually decreases toward their mouths. Ungraded streams have steep sections at various points, and typically have rapids and waterfalls at numerous locations along their lengths.
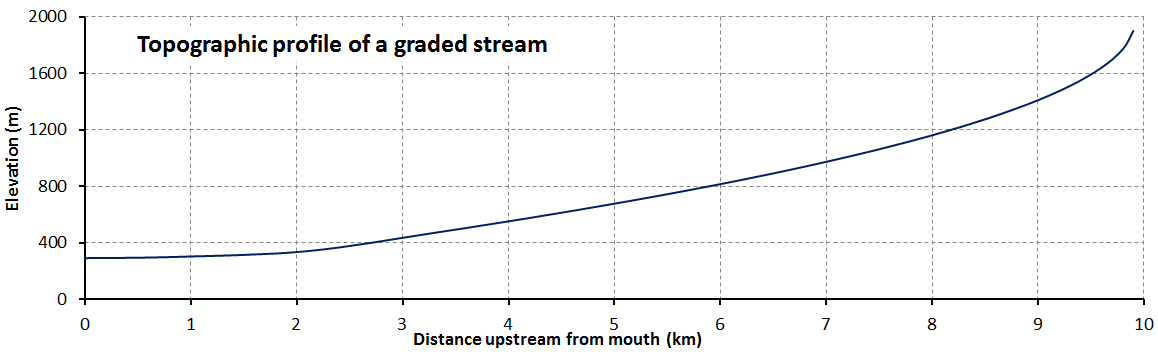
A graded stream can become ungraded if there is renewed tectonic uplift, or if there is a change in the base level, either because of tectonic uplift or some other reason. As stated earlier, the base level of Cawston Creek is defined by the level of the Similkameen River, but this can change, and has done so in the past. Figure 13.2.7 shows the valley of the Similkameen River in the Keremeos area. The river channel is just beyond the row of trees. The green field in the distance is underlain by material eroded from the hills behind and deposited by a small creek (not Cawston Creek) adjacent to the Similkameen River when its level was higher than it is now. Sometime in the past several centuries, the Similkameen River eroded down through these deposits (forming the steep bank on the other side of the river), and the base level of the small creek was lowered by about 10 metres. Over the next few centuries, this creek will seek to become graded again by eroding down through its own alluvial fan.

Another example of a change in base level can be seen along the Juan de Fuca Trail on southwestern Vancouver Island. As shown in Figure 13.2.8, many of the small streams along this part of the coast flow into the ocean as waterfalls. It is evident that the land in this area has risen by about 5 metres in the past few thousand years, probably in response to deglaciation. The streams that used to flow directly into the ocean now have a lot of down-cutting to do to become regraded.
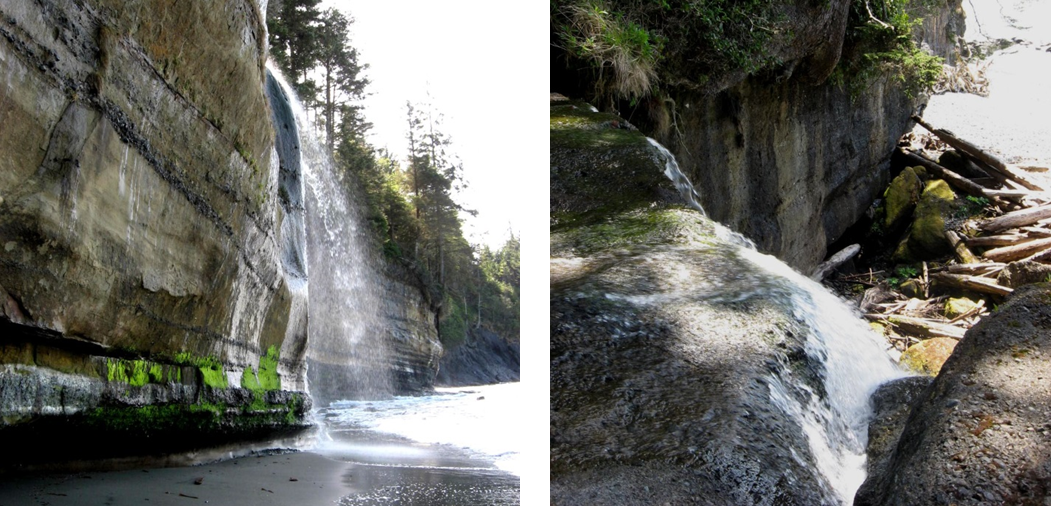
The ocean is the ultimate base level, but lakes and other rivers act as base levels for many smaller streams. We can create an artificial base level on a stream by constructing a dam, as illustrated in Exercise 13.2.
Exercise 13.2 The effect of a dam on base level
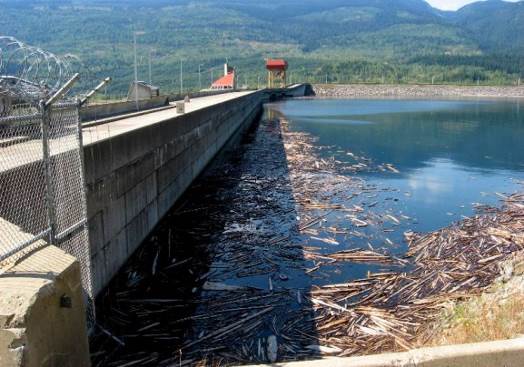
When a dam is built on a stream, a reservoir (artificial lake) forms behind the dam. This temporarily (for many decades at least) creates a new base level for the part of the stream above the reservoir.
How does the formation of a reservoir affect the stream where it enters the reservoir, and what happens to the sediment it was carrying?
The water leaving the dam has no sediment in it. How does this affect the stream below the dam?
See Appendix 3 for Exercise 13.2 answers.
Sediments accumulate within the flood plain of a stream, and then, if the base level falls, or if there is less sediment to deposit, the stream may cut down through those existing sediments to form terraces. A terrace on the Similkameen River is shown in Figure 13.2.7 and some on the Fraser River are shown in Figure 13.2.10. The Fraser River photo shows at least two levels of terraces.
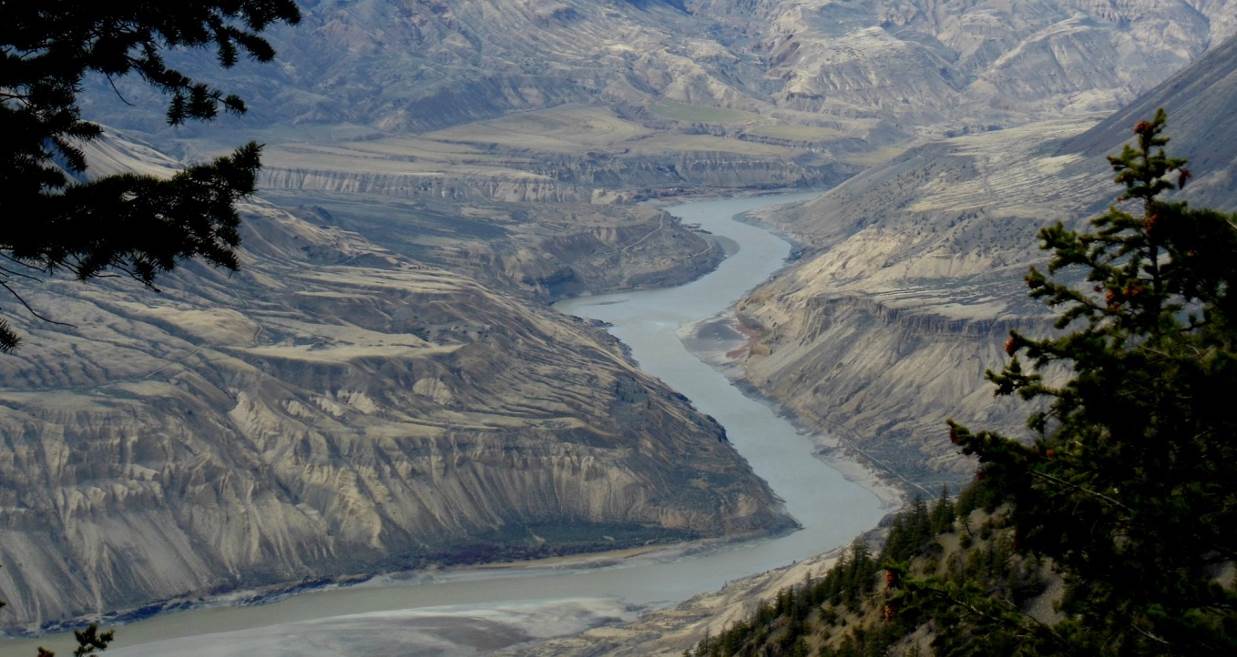
In the late 19th century, American geologist William Davis proposed that streams and the surrounding terrain develop in a cycle of erosion (Figure 13.2.11). Following tectonic uplift, streams erode quickly, developing deep V-shaped valleys that tend to follow relatively straight paths. Gradients are high, and profiles are ungraded. Rapids and waterfalls are common. During the mature stage, streams erode wider valleys and start to deposit thick sediment layers. Gradients are slowly reduced and grading increases. In old age, streams are surrounded by rolling hills, and they occupy wide sediment-filled valleys. Meandering patterns are common.
Davis’s work was done long before the idea of plate tectonics, and he was not familiar with the impacts of glacial erosion on streams and their environments. While some parts of his theory are out of date, it is still a useful way to understand streams and their evolution.
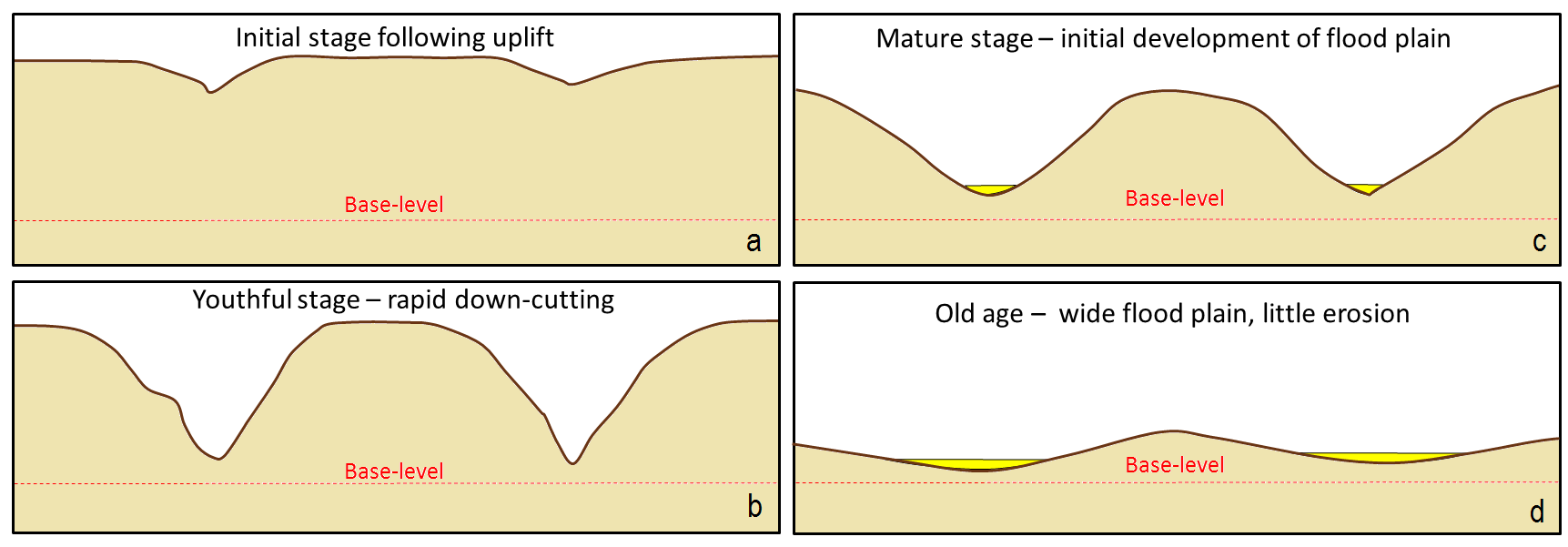
Image Descriptions
Figure 13.2.1 image description: Cawston Creek is a drainage basin made up of a number of small streams that flow into the Similkameen River. The Cawston Creek drainage basin also has a smaller tributary drainage basin that flows into it. [Return to Figure 13.2.1]
Figure 13.2.2 image description: The graph shows the topographical profile of the Cawston Creek channel. Starting at 10 kilometres from the Similkameen River, the channel is at an elevation of 1800 metres. It flows downhill towards the river at a steady decline for seven kilometres. At three kilometres from the Similkameen River at an elevation of 275 metres, the channel levels out and stays at that elevation until it joins with the Similkameen River. This is conveyed in the following table.
Distance from the Similkameen River (kilometres) | Elevation (metres) |
---|---|
10 | 1,800 |
9 | 1,750 |
8 | 1,500 |
7 | 1,300 |
6 | 1,125 |
5 | 950 |
4 | 600 |
3 | 280 |
2 | 275 |
1 | 275 |
0 | 275 |
To determine the gradient of the stream, you must divide the change in elevation (rise) by the change in distance (run). For example, the elevation at 4.5 kilometres from the river is 800 metres and at 8.0 kilometres from the river, the elevation is 1500 metres. That means that in 3.5 kilometres (run), elevation increased by 700 metres (rise). To determine the gradient, you must divide rise (700 metres) by run (3.5 kilometres), which equals a vertical increase of 200 metres per kilometre.
Figure 13.2.4 image description: Three types of drainage patterns:
- Dendritic: a pattern of drainage channels that resembles the branches in a tree.
- Trellis: a drainage pattern in which tributaries typically flow parallel to one other but meet at right angles.
- Rectangular: a drainage pattern in which tributaries typically flow at right angles to each other and meet at right angles.
Figure 13.2.5 image description: Two types of drainage patterns:
- Deranged: a pattern of drainage channels that is chaotic.
- Radial: a pattern of streams radiating out from a central point, typically an isolated mountain.
Figure 13.2.6 image description: The topographic profile of a typical graded stream. While the topographical profile of Cawston Creek fell at a steady rate and then leveled out for the last three kilometres, the topographical profile of a typical graded stream starts out steep, but the slope (or gradient) gradually decreases towards the bottom to form a soft curve. [Return to Figure 13.2.6]
Figure 13.2.10 image description: The Fraser River snaking through a channel that it has worn down over the years. The sides of the bank rise steeply, level out, and then rise again to produce terraces. [Return to Figure 13.2.10]
Figure 13.2.11 image description: William Davis’s cycle of erosion.
- Initial stage: A tectonic uplift forms two sharp valleys on each side.
- Youthful stage: Steams flowing through those valleys erode the surrounding earth quickly and cut deep to form sharp V’s.
- Mature stage: The streams become wider and the banks more gradual. This marks the initial development of a flood plain.
- Old age: Steams for a wide flood plain where little erosion occurs.
Media Attributions
- Figures 13.2.1, 13.2.2, 13.2.4, 13.2.5, 13.2.6, 13.2.7, 13.2.8, 13.2.9, 13.2.11: © Steven Earle. CC BY.
- Figure 13.2.3: Image by Metro Vancouver. Used with permission.
- Figure 13.2.10: Image by Marie Betcher. Used with permission.
Volcanic Hazards
Volcanoes are responsible for a large number of deaths, but lava is not the only danger associated with these hazards. Mount Vesuvius (Naples, Italy) is infamous for its violent explosion thousands of years ago in 79 AD when a pyroclastic flow travelled over the Roman countryside and engulfed the cities of Pompeii and Herculaneum [1]. It was not until the 18th century that we uncovered the shocking remains of these towns beneath over 10 feet of ash and the casts of people preserved within it.
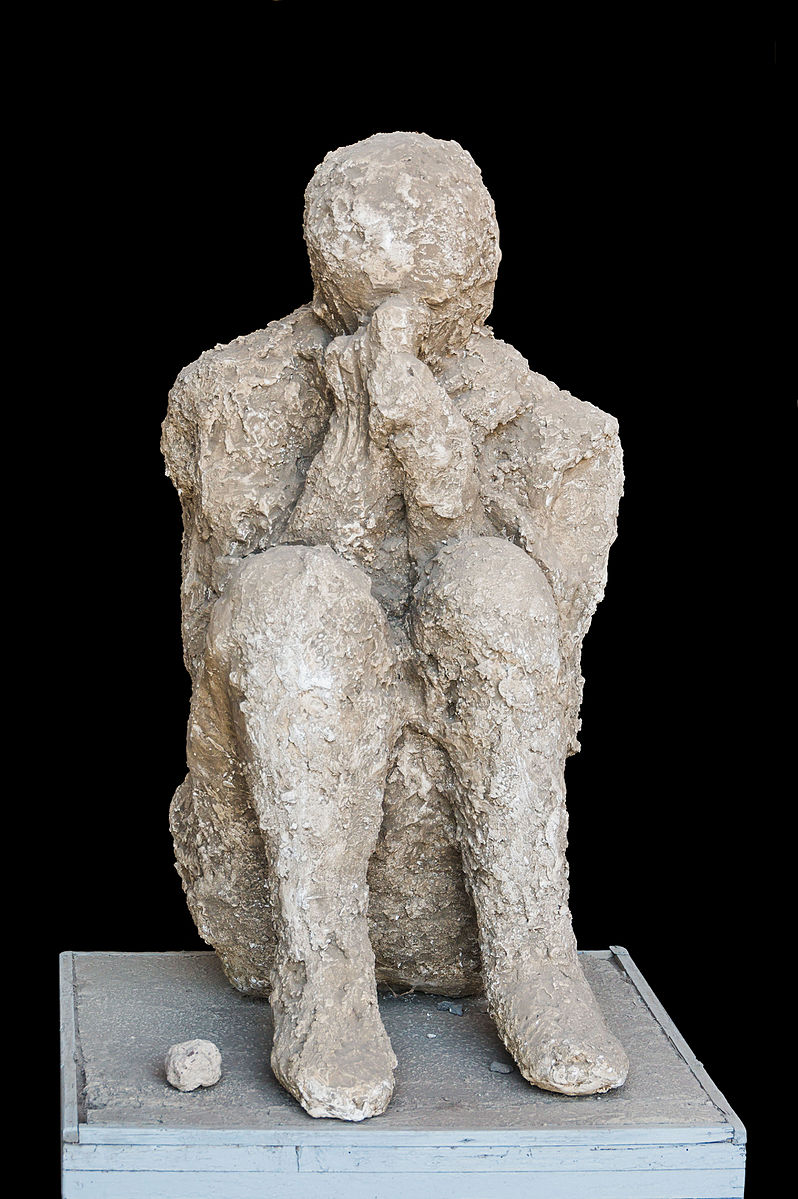
We know more about volcanic hazards over the past century because they have been better monitored and documented. We have seen enormously violent explosions, effusive lava, fast pyroclastic flows, ash, landslides, toxic gases, and more!
Pyroclastic Flows
The most dangerous type of volcanic hazard are pyroclastic flows. These flows are a mix of lava blocks, pumice, ash, and hot gases between 400 to 1,300°F! The turbulent cloud of ash and gas races down the steep flanks at high speeds at an average of 60 mph (much faster than people can run) into the valleys where farmlands grow and cities thrive [1].

Pyroclastic flows can often be expected of stratovolcanoes that contain felsic or intermediate magma. This magma is silica-rich and contains volatile gases that make it highly viscous. Therefore, these volcanoes often have very violent eruptions that are accompanied by a pyroclastic flow.
There are numerous examples of deadly pyroclastic flows. In 2014, the Mount Ontake pyroclastic flow in Japan killed 47 people. The flow was caused by magma heating groundwater into steam, which then rapidly ejected with ash and volcanic bombs. Some were killed by inhalation of toxic gases and hot ash, while volcanic bombs struck others [1]. In 1902, on the Caribbean Island Martinique, Mount Pelee erupted with a violent pyroclastic flow that destroyed the entire town of St. Pierre and killing 28,000 people in moments [3].
Lahars
https://www.youtube.com/watch?v=y9080YePWKY
A lahar is an Indonesian word for a mudflow that is a mixture of water, ash, rock fragments, and other debris that moves down the mountainside of a volcano (or other nearby mountains covered with freshly-erupted ash). They form from the rapid melting of snow or glaciers on volcanoes or sometimes in combination with a new eruption and heavy thunderstorm, as seen at Mt. Pinatubo.
Lahars move like a slurry of concrete, but they can move extremely fast at speeds up to 50 mph. Part of the reason they are so deadly is because they are slurry-like; they easily capture materials in their wake and they can travel very long distances like a flash flood [1].
During the 1980 Mount St. Helens eruption, lahars reached 17-miles (27 km) down the North Fork of the Toutle River. Another scenario played out when a lahar from the volcano Nevado del Ruiz Colombia, buried a town in 1985 and killed an estimated 25,000 people [1].

Tephra and Ash
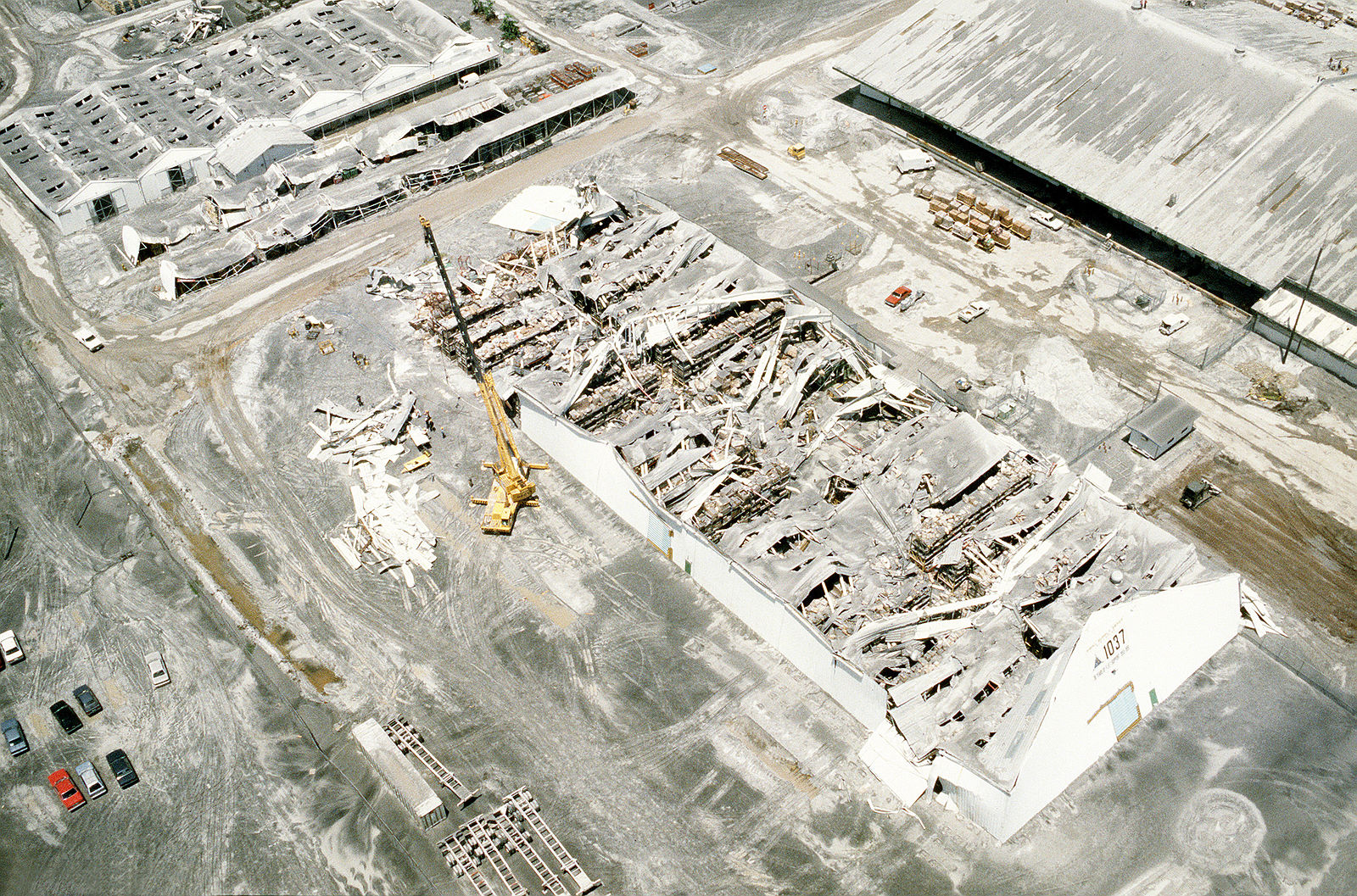
Volcanoes—mainly stratovolcanoes—eject substantial amounts of tephra and ash [1].
Tephra is heavier than ash, so it will fall closer to the volcano's crater and vent. Large masses of tephra sometimes erupt from volcanoes and can pose deadly hazards to anyone nearby. These are called volcanic bombs [1].

Ash is much finer, but it should not be underestimated. It can be carried much longer distances away from the volcano, and that can cause more widespread issues in nearby towns and cities. A build-up of ash can collapse the roof of a building or home, and the microscopic minerals within ash will cause respiratory illnesses such as silicosis. Inhaling ash is extremely hazardous because much of it contains microscopic volcanic glass particles [4]. Imagine inhaling tiny shards of glass!
Ash will also interfere with transportation services farther away from an eruption. For example, the 2010 Eyjafjallajökull volcanic eruption in Iceland created a gigantic ash cloud that caused a significant air travel disruption in northern Europe. No one was hurt, but the cost to the world economy was estimated to be billions of dollars [3].
Volcanic Gases
Magma contains dissolved volatile gases. As magma rises toward the surface, the pressure that keeps it in

the magma chamber will start to decrease, which allows those gases to escape. Think of this process like twisting the cap of a soda bottle; the first thing to rise and escape in that initial hissing noise is gas [1]!
The types of gases that are commonly released from volcanoes include greenhouse gases such as carbon dioxide (CO2), sulfur dioxide (SO2), hydrogen sulfide (H2S), methane (CH4), and water vapor (H2O). There are also toxic and acidic gases present at volcanoes such as HF, HBr, and HCl. After gigantic volcanic explosions, some volcanic gases such as sulfur dioxide will become sulfate aerosols in the atmosphere. These aerosol particles block sunlight coming toward Earth's surface and cause the planet to become cooler [5].
The volcanic gases can be both toxic and suffocating, and these gases sometimes are released from a volcano without an accompanying eruption. Gases were released from the Oku Volcanic Plain in Lake Nyos, Cameroon, and the carbon dioxide suffocated almost 2,000 people in 1986 [1].
a pattern in which temperatures in the crust increase by about 25 degrees Celsius per kilometer (km) of depth.
Surface Currents
Ocean water moves in predictable ways along the ocean surface. Surface currents can flow for thousands of kilometers and can reach depths of hundreds of meters. These surface currents do not depend on the weather; they remain unchanged even in large storms because they depend on factors that do not change. (2)
https://www.youtube.com/watch?v=6vgvTeuoDWY&feature=emb_logo
Surface currents are created by three things: global wind patterns, the rotation of the earth, and ocean basins' shape. Surface currents are significant because they distribute heat around the planet and are a significant factor influencing climate around the globe.
Global Wind Currents
Winds on Earth are either global or local. Global winds blow in the same directions all the time and are related to the unequal heating of Earth by the Sun, that is that more solar radiation strikes the equator than the polar regions, and the rotation of the Earth called the Coriolis effect. The causes of the global wind patterns will be described later when we look at the atmosphere. (2) Water in the surface currents is pushed in the direction of the significant wind belts:
- Trade winds are consistent winds that flow east to west between the equator and 30 degrees North and 30 degrees South latitude.
- Westerlies are the prevailing winds that blow from the west in the middle latitudes.
- Polar easterlies are winds that flow from the east between 50 degrees and 60 degrees north and south latitude, as well as the north and south poles.
Rotation of the Earth
The wind is not the only factor that affects ocean currents. The Coriolis effect describes how Earth's rotation steers winds and surface ocean currents. The Coriolis effect causes freely moving objects to appear to move to the right in the Northern Hemisphere and to the left in the Southern Hemisphere. The objects themselves are moving straight, but the Earth is rotating beneath them, so they seem to bend. (2)
https://www.youtube.com/watch?v=xusdWPuWAoU&feature=emb_logo
An example might make the Coriolis effect easier to visualize. If an airplane flies five hundred miles due north, it will not arrive at the city that was due north of it when it began its journey. Over the time it takes for the airplane to fly five hundred miles, that city moved, along with the Earth it sits on. Therefore, the airplane will arrive at a city to the west of the original city (in the Northern Hemisphere) unless the pilot has compensated for the change. So, to reach his intended destination, the pilot must also veer right while flying north.
As wind or ocean currents move, the Earth spins underneath it. As a result, an object moving north or south along the Earth will appear to move in a curve, instead of in a straight line. Wind or water that travels toward the poles from the equator is deflected to the east, while wind or water that travels toward the equator from the poles gets bent to the west. The Coriolis effect bends the direction of surface currents to the right in the Northern Hemisphere and left in the Southern Hemisphere. (2)
Deep Currents
Thermohaline circulation drives deep ocean circulation. Thermo means heat, and haline refers to salinity. Differences in temperature and salinity change the density of seawater. Thermohaline circulation is the result of density differences in water masses because of their different temperature and salinity. (5)

Lower temperature and higher salinity yield the densest water. When a volume of water is cooled, the molecules move less vigorously, so the same number of molecules takes up less space, and the water is denser. If salt is added to a volume of water, there are more molecules in the same volume, so it is denser. Changes in temperature and salinity of seawater take place at the surface. Water becomes dense near the poles. Cold polar air cools the water and lowers its temperature, increasing its salinity. Freshwater freezes out of seawater to become sea ice, which also increases the salinity of the remaining water. This frigid, very saline water is very dense and sinks, a process called downwelling. (1)
Two things then happen. The dense water pushes deeper water out of its way, and that water moves along the bottom of the ocean. This deep-water mixes with less dense water as it flows. Surface currents move water into the space vacated at the surface where the dense water sank. Water also sinks into the deep ocean off Antarctica. Since unlimited amounts of water cannot sink to the ocean's bottom, water must rise from the deep ocean to the surface somewhere. This process is called upwelling. (1)
https://www.youtube.com/watch?v=1uuqvu--6Yg&feature=emb_logo
Upwelling occurs along the coast when the wind blows water strongly away from the shore. This leaves a void that is filled with deep water that rises to the surface. Upwelling is significant where it occurs. During its time on the bottom, the cold deep water has collected nutrients that have fallen through the water column. Upwelling brings those nutrients to the surface. That nutrient supports the growth of plankton and forms the base of a vibrant ecosystem. California, South America, South Africa, and the Arabian Sea all benefit from offshore upwelling. Upwelling also takes place along the equator between the North and South Equatorial Currents. Winds blow the surface water north and south of the equator, so deep water undergoes upwelling. The nutrients rise to the surface and support a great deal of life in the equatorial oceans. (2)
Our "Contracting Earth"?
How can we explain the movement of land around us over long periods of time? Sometimes volcanoes spring up when there were previously none. Other times, the ground shakes in certain places. Some areas, like Arizona, are home to amazing mountain ranges and valleys, and others are island destinations. How did we make sense of any of this?
Science is an evolving thing, which means that scientists don't always get it right. Case in point: up until the mid-1900s we believed our planet formed all its breath-taking features by "squeezing" them out! These scientists rightly believed that Earth was a ball of hot, molten material when it first formed in space. Where they went wrong, however, is the belief that the cooling outer shell, contracted like a dried-out raisin [1].
https://www.youtube.com/watch?v=8Yn2KvRVr28
Furthermore, these scientists thought that new landscapes formed through a process called isostasy. This process involved the continents and ocean basins sinking and rising up as they experienced changes in density [1].
https://www.youtube.com/watch?v=t2CFSuvm1A4
Continental Drift
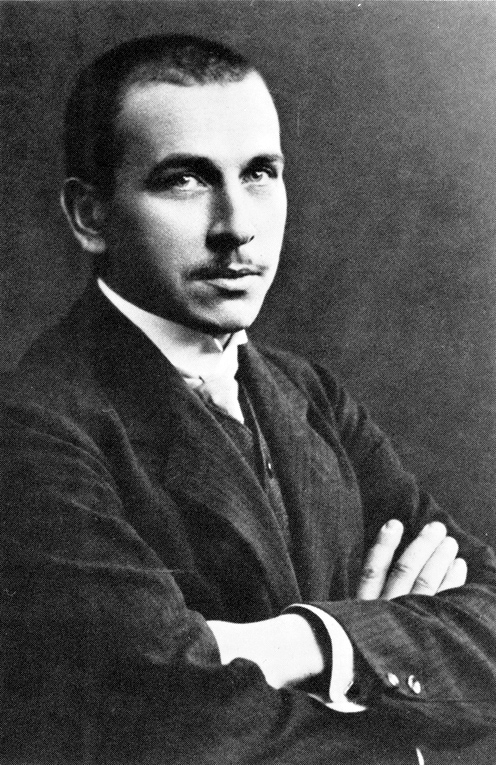
Alfred Wegener (1880-1930) was a German scientist who specialized in meteorology and climatology. He enjoyed field work in Greenland to establish weather monitoring stations and made great contributions to climatology. However, as an avid explorer of the planet, he had something to say about how it operated. In 1910, he publicly disagreed with the extent that the role of isostasy played in the Contracting Earth hypothesis. He furthermore noticed some patterns in our world map that led him to propose a radical counter-hypothesis: the concept of Continental Drift. [2]
https://www.youtube.com/watch?v=ttg5fZk3ddE
Ever since the first world map, people have noticed the similarities between the coastlines of South America and Africa. Would it be such a stretch to imagine these continents being pushed together like two pieces of a jigsaw puzzle? Indeed, what if all of the continents were once merged together at one point as a single landmass, and they broke apart for some reason?
The idea that South America and Africa were once attached as a single landmass was not a new one; Antonio Snider-Pellegrini even did preliminary work on continental separation and matched fossils between the two continents in 1858 [2]. But Wegener's contribution to this idea was new and interesting in that he collected a tremendous amount of data on these two continents. Let's review some of this evidence below!
Fossils
The fossils of the ancient life that existed hundreds of millions of years ago have been found along the separate coastlines of not just Africa and South America, but also India, Australia, and Antarctica (see left figure)! These organisms appeared to be exactly the same, but how could they have lived on separate continents? For example, neither the reptile Mesosaurus nor Cynognathus, which were found in South America and Africa could live in salty ocean water. Therefore, Wegener argued, these animals could not have crossed the ocean to live on the continents if they were always separated. That meant that at one time, the continents had to have been merged together as a single landmass!
Those that disagreed with Wegener's hypothesis made this counter argument: perhaps the continents were in the same configurations hundreds of millions of years ago as they are today. Instead of swimming across deep oceans, these ancient reptiles might have traveled between the continents on narrow land bridges that stretched across the oceans [1]. The only reason we don't see these land bridges today is because they have sunk down or eroded away.
Which idea makes more sense to you: the continents once being merged together OR the idea of ancient land bridges? Science is about a healthy debate given data and evidence, but Wegener was not done!
Geologic Evidence
Even if ancient reptiles and animals crossed the oceans on land bridges, as Wegener's opponents argued, there was something that they could not explain: the Geologic Record.
Think of a volcanic eruption, a landslide, a winding river, or a sandy beach. When a layer of rock is deposited, it reflects an environment or event that has happened in the area. Each layer of rock is like a page in a book that each of us can learn to read. Therefore, if one area has a distinct sequence of layers, a trained geologist can tell you that region's unique history. Let's keep this "book" metaphor in mind.
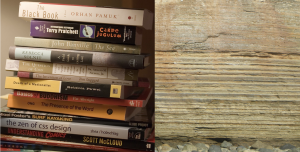
When Wegener examined the layers of rock between two separate continents, he found something exciting. Places an ocean apart from each other, such as the Appalachians in the United States and the mountains extending through Greenland, Ireland, the United Kingdom, and Norway had the same sequence of rocks. That is essentially finding the same book two continents apart.
What would a reasonable person conclude when finding the same book with two different covers? The same author wrote it. Much in the same spirit, Wegener concluded that these two mountain ranges in North America and Europe actually formed as one single chain hundreds of millions of years ago when these two continents were merged together. North America and Europe then drifted apart to the place we see them now on the world map.
Climatic Evidence
Let's not forget that Wegener was a climatologist, and as he traveled the continents, he began to notice some strange patterns in the geologic record. Wegener found indications that places like southern Africa, India, Australia, and the Arabian subcontinent were once glaciated about 250 million years ago. He also discovered fossils of tropical plants in areas north of the Arctic Circle. In short, the rocks and fossils Wegener observed were telling him that places that we know today are cold were once hot, and places that are hot were once cold.
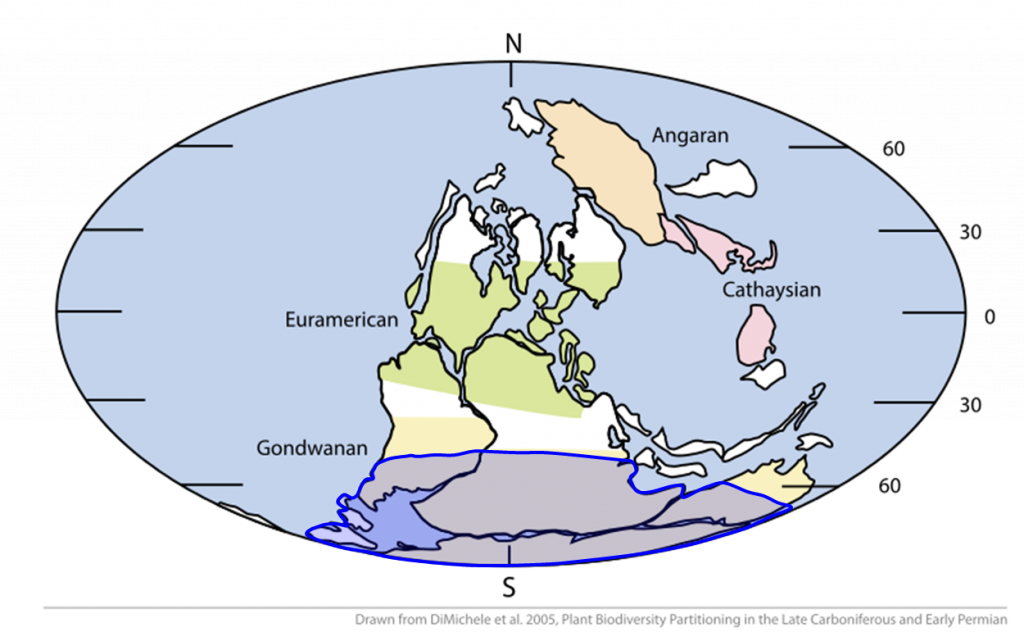
Wegener used these observations to further conclude that the continents must have moved. For example, Wegener knew that glaciers only formed near the poles in the modern day climate; therefore, he argued that to have glaciers, India, Australia, and Africa must have once been centered around the south pole. Similarly, today's Arctic Circle must have once been around the tropics - approximately 23 °N and S in along Earth's latitude to host tropical plant life [3].
Putting the Puzzle Together
After gathering a significant amount of evidence across the world, Alfred Wegener took the bold step of publishing his Continental Drift hypothesis in 1915 in a book entitled Die Entstehung der Kontinente und Ozeane or "The Origin of Continents and Oceans". In this book, Wegener presents all of his evidence, and asserts that the continents must have once been together in a single landmass called "Ur kontinent" (supercontinent) or "Pangäa" (entire earth). Over large timescales of millions of years, this landmass broke apart, and the continents shifted into the configuration as we know them today.
https://www.youtube.com/watch?v=O5jQLKWvYBA
In order to successfully argue for Continental Drift, Wegener also had the monumental task of dismantling the case for the Contracting Earth hypothesis and the popular idea that all ancient life moved across separate continents using narrow land bridges. In his book, Wegener makes the following appeal:
Backlash and Legacy
How Bad Was the Criticism Against Wegener?
“delirious ravings”
“moving crust disease and wandering pole plague.”
“Germanic pseudo-science”
“wrong for a stranger to the facts he handles to generalize from them"
“utter damned rot”
Alfred Wegener's Final Expedition
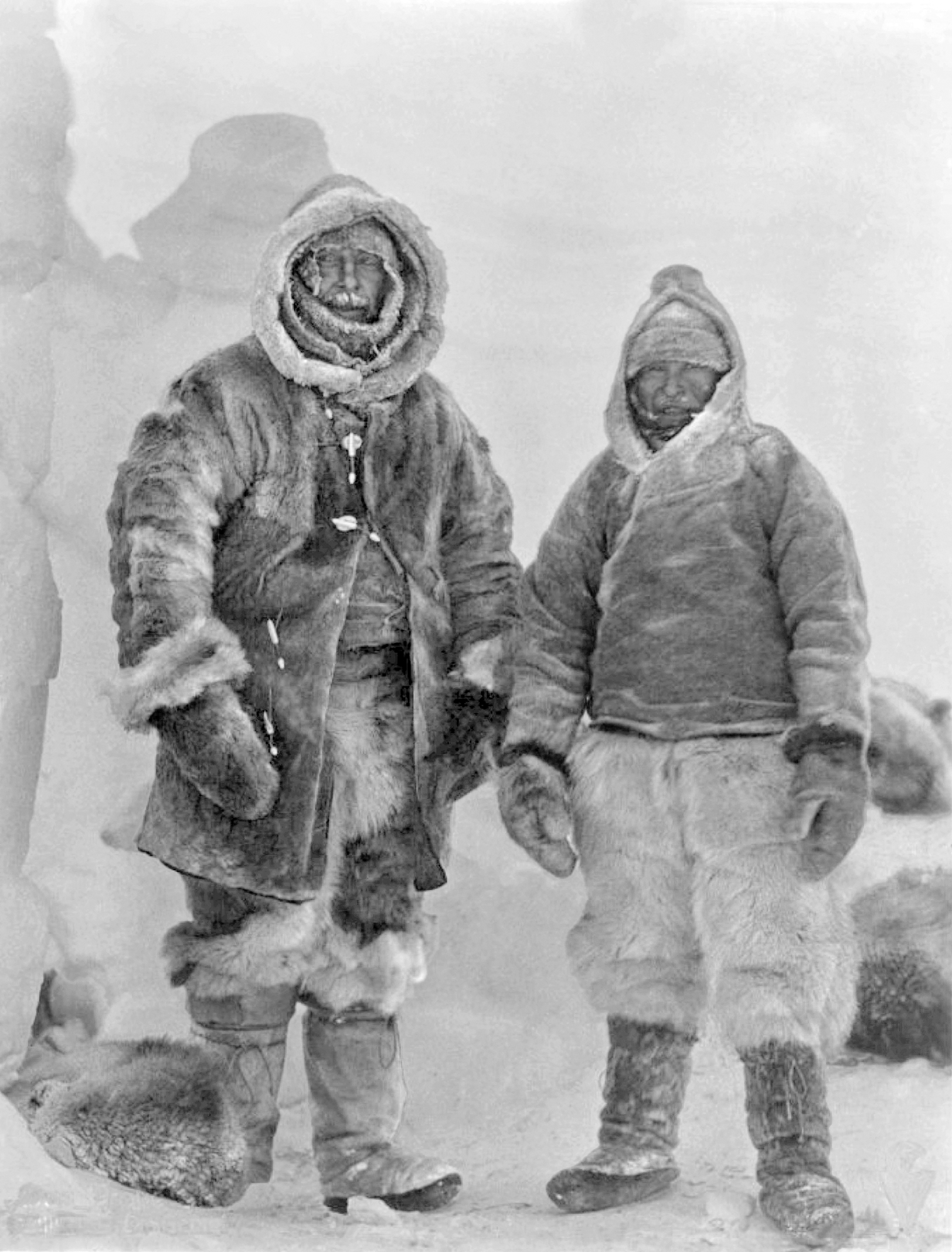
Wegener’s scientific contributions were not only in the field of geosciences. As a climatologist, one of his passions was exploring the north of Greenland, which in the early 1900s had not been completely mapped. Wegener helped establish weather-monitoring stations on Greenland that took many readings of its brutal winter weather. This project became a lifelong love for Wegener despite the deadly risks each expedition posed. In 1928, even though Wegener was approaching his 50s, he departed again to explore northern Greenland. The weather was particularly bad (-76 degrees F) and one of the nearby camps was running low on food. He managed to travel through the terrible weather to resupply the camp on dog sleds, but the success was short-lived. Even with supplies, it was clear that the food would not last long enough for everyone. Therefore, he and another colleague volunteered to go back into the weather in the hopes of traveling to another camp. Wegener died on the journey from a heart attack, and his grave was marked with skis. His brother eventually discovered his body, but the family agreed that Wegener belonged in the field he so loved, and his final resting place has since remained Greenland [5,6].
Divergent boundaries are places where two or more plates move away from each other. These boundaries can be found on continental or oceanic lithosphere. Tensional stress operates between the tectonic plates at a divergent boundary, which causes the lithosphere at these locations to stretch and pull apart. Divergent boundaries slowly grow ocean basins along continental lithosphere.
The process of divergence is responsible for breaking up continental landmasses and supercontinents. This boundary type is the reason why the generations of scientists noticed the apparent fit between the coastlines of South America and Africa. Indeed, it is the reason that the world map appears the way it does today!
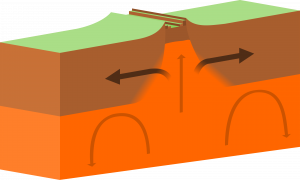
Continental Rift Valleys
How does a continent, or even a supercontinent, break apart? At first glance, such a task seems difficult considering continental lithosphere can be up to 20 times thicker than oceanic lithosphere due to billions of years of mountain-building. However, the insulation from that thick lithosphere will eventually allow a hot plume of magma from the mantle to rise toward the Earth's surface. That mantle plume will weaken the continental lithosphere, and eventually the convection cells within the mantle will be able to push the continent apart.
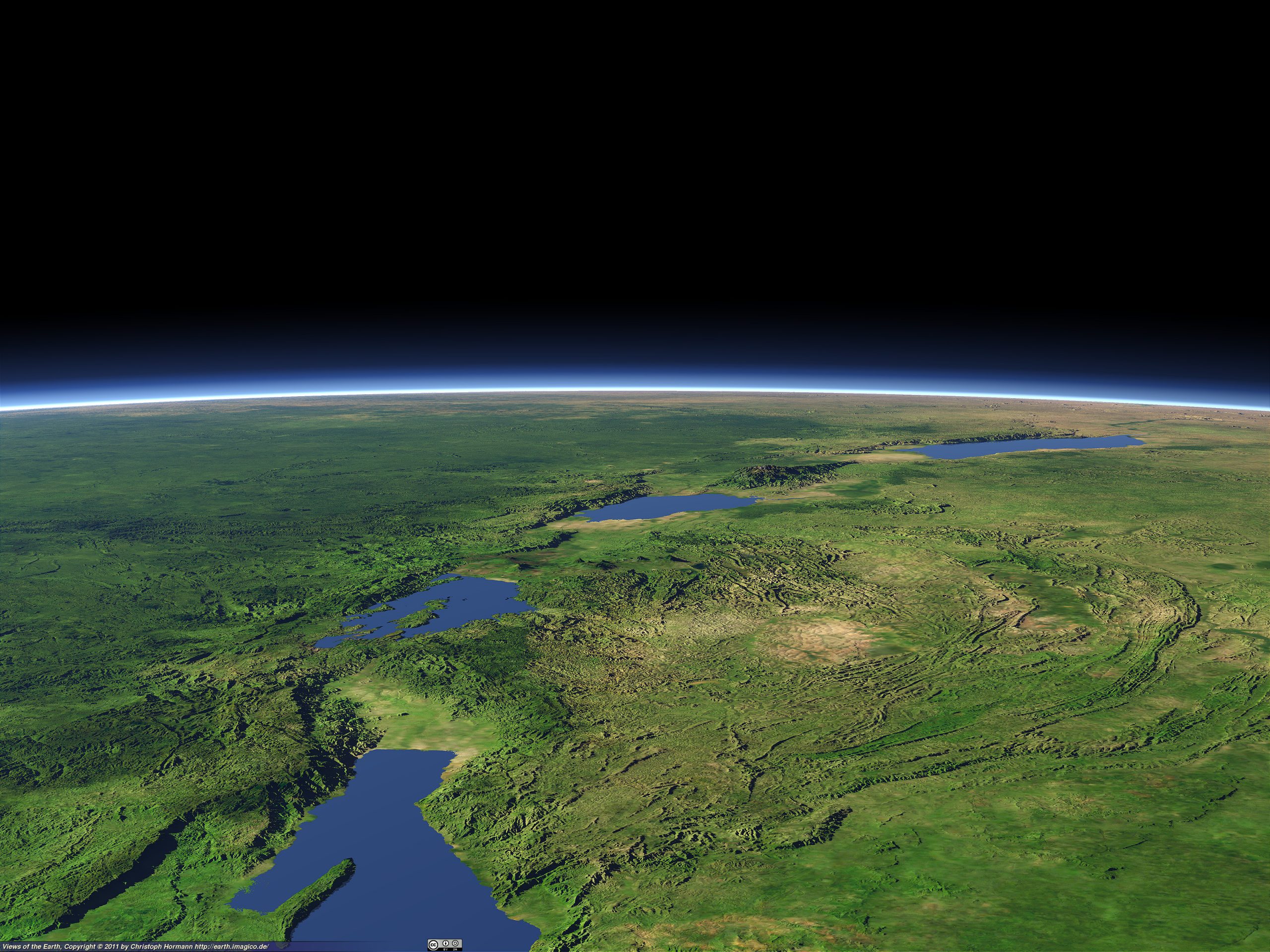
A continental rift valley is a region where the continental lithosphere is weakening and stretching apart. It is an observable divergent boundary and a sign that a continent is breaking apart. Given enough time, the rifting will continue to a point in which the continental lithosphere will become so thin, it will become predominantly enriched in the mantle plume materials. At that point, the rift will have created brand new oceanic crust, and a newborn ocean basin will open between the continental fragments.
This process of continental rifting has taken place about 250 million years ago between South America and Africa, as well as North America and Eurasia during the breakup of the supercontinent Pangaea. From a single landmass, the Earth saw many new continents form as well as a narrow sea that would one day widen to become our Atlantic Ocean.
https://www.youtube.com/watch?v=pf5KPXP4exc
PLATE TECTONICS IN ACTION: CONTINENTAL RIFT VALLEYS
Some of the world's continents are breaking apart as we speak! Here are some examples of regions undergoing continental rifting:
- The East African Rift Valley (Djibouti, Ethiopia, Uganda, Kenya, Tanzania)
- The Red Sea (Egypt, Sudan, Eritrea, Djibouti, Saudi Arabia, Yemen)
- Lake Baikal (Russia)
Mid-Ocean Ridges
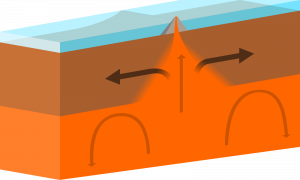
We have previously discussed the discovery of Mid-Ocean Ridges as a key piece of evidence for the theory of plate tectonics. The reason these spreading centers along the ocean floor are essential for plate tectonics is that they represent a clear example divergent boundary.
Mid-Ocean Ridges typically follow the long process of continental rifting. Once continental lithosphere has thinned and become mafic, upwelling magma begins to produce new oceanic lithosphere and a narrow ocean basin forms between the rifted continents (review video in previous section). As this process evolves, a Mid-Ocean Ridge forms above the rift on the floor of the new ocean because the cooling lava is hot and less dense (i.e., it takes up more space than cold material).
The places where we find Mid-Ocean Ridges are called spreading centers. These are sometimes characterized as "conveyer-belts" because they are the one site on the planet where new oceanic lithosphere is constantly created. These spreading centers are volcanoes; molten magma from the asthenosphere erupts underwater as lava and rapidly cools as new oceanic lithosphere. The material near the spreading centers is relatively hot and less dense, and therefore it has a higher elevation. Mid-Ocean Ridges often form long, high-elevation mountain chains because of their underwater volcanism.
Some Like It Boiling: Life at Hydrothermal Vents
Mid-ocean ridges also are home to some of the unique ecosystems discovered, found around hydrothermal vents that circulate ocean water through the shallow oceanic crust, and send it back out to rich chemical compounds and heat. While it was known for some time that hot fluids could be found on the ocean floor, it was only in 1977 when a team of scientists using the Diving Support Vehicle Alvin discovered a thriving community of organisms, including tubeworms bigger than people!
https://www.youtube.com/watch?v=R1koFEKfmLw
This group of organisms is dependent on the sun and photosynthesis but instead relies on chemical reactions with sulfur compounds and heat from within the Earth, a process known as chemosynthesis. Before this discovery, the thought in biology was that the sun was the ultimate source of energy in ecosystems; now, we know this to be false. Not only that, but some have also suggested it is from this that life could have started on Earth, and it now has become a target for extraterrestrial life (e.g., Jupiter's moon Europa).
Continued volcanism will cause the new oceanic lithosphere to move away from the Mid-Ocean Ridges, and that lithosphere will cool and sink deeper along the ocean floor. Because new oceanic lithosphere is always produced at a spreading center, the age of the seafloor is predictably younger near a Mid-Ocean Ridge and older as it moves farther away.
Divergent plate motion at a Mid-Ocean Ridge occurs at different rates, depending on the location. Spreading can be as fast as 20 cm/year or as slow as 1 cm/year. At these rates over hundreds of millions of years, it is not a surprise that the continents have moved so far apart.
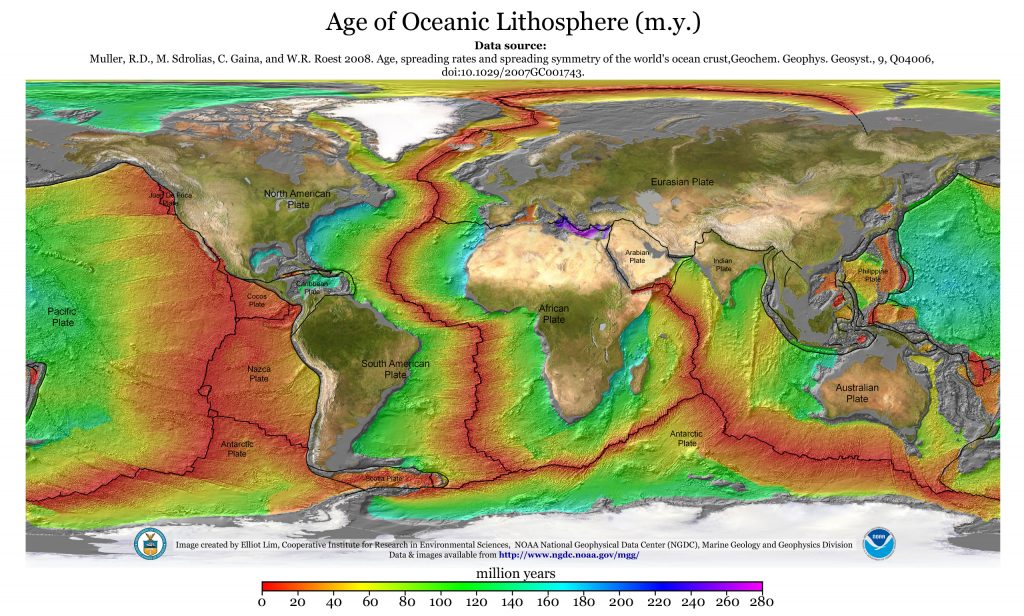
PLATE TECTONICS IN ACTION: MID-OCEAN RIDGES
Although they are sometimes deep underwater, we have known about mid-ocean ridges for decades! Here are some good examples of this type of divergent boundary:
- The Mid-Atlantic Ridge
- The East Pacific Rise
- The Gakkel Ridge/ Mid-Arctic Ridge
As has already been described, igneous rocks are classified into four categories: felsic, intermediate, mafic, and ultramafic, based on either their chemistry or their mineral composition. The diagram in Figure 4.4.1 can be used to help classify igneous rocks by their mineral composition. An important feature to note on this diagram is the red line separating the non-ferromagnesian silicates in the lower left (K-feldspar, quartz, and plagioclase feldspar) from the ferromagnesian silicates in the upper right (biotite, amphibole, pyroxene, and olivine). In classifying intrusive igneous rocks, the first thing to consider is the percentage of ferromagnesian silicates. In most igneous rocks the ferromagnesian silicate minerals are clearly darker than the others, but it is still quite difficult to estimate the proportions of minerals in a rock.
Based on the position of the red line in Figure 4.4.1, it is evident that felsic rocks can have between 1% and 20% ferromagnesian silicates (the red line intersects the left side of the felsic zone 1% of the distance from the top of the diagram, and it intersects the right side of the felsic zone 20% of the distance from the top). Intermediate rocks have between 20% and 50% ferromagnesian silicates, and mafic rocks have 50% to 100% ferromagnesian silicates. To be more specific, felsic rocks typically have biotite and/or amphibole; intermediate rocks have amphibole and, in some cases, pyroxene; and mafic rocks have pyroxene and, in some cases, olivine.
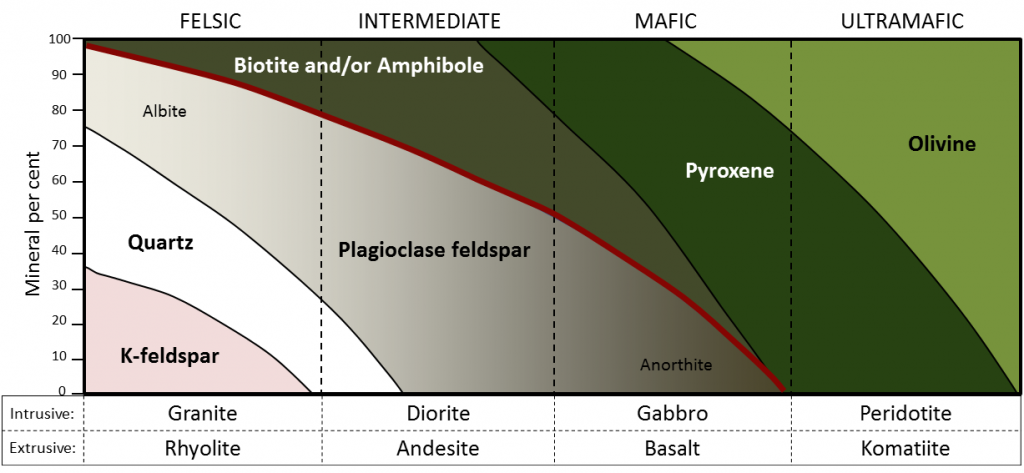
If we focus on the non-ferromagnesian silicates, it is evident that felsic rocks can have from 0% to 35% K-feldspar, from 25% to 35% quartz (the vertical thickness of the quartz field varies from 25% to 35%), and from 25% to 50% plagioclase (and that plagioclase will be sodium-rich, or albitic). Intermediate rocks can have up to 25% quartz and 50% to 75% plagioclase. Mafic rocks only have plagioclase (up to 50%), and that plagioclase will be calcium-rich, or anorthitic.
Exercise: Mineral proportions in igneous rocks

Figure 3.4.3 provides a diagrammatic representation of the proportions of dark minerals in light-coloured rocks. You can use that when trying to estimate the ferromagnesian mineral content of actual rocks, and you can get some practice doing that by completing Exercise 3.6. Be warned! Geology students almost universally over-estimate the proportion of dark minerals.

Exercise 3.6 Proportions of ferromagnesian silicates
The four igneous rocks shown below have differing proportions of ferromagnesian silicates. Estimate those proportions using the diagrams in Figure 3.4.3, and then use Figure 3.4.1 to determine the likely rock name for each one.
![]() |
![]() |
![]() |
![]() |
___% | ___% | ___% | ___% |
__________ | __________ | __________ | __________ |
See Appendix 3 for Exercise 3.6 answers.
Igneous rocks are also classified according to their textures. The textures of volcanic rocks will be discussed in Chapter 4, so here we’ll only look at the different textures of intrusive igneous rocks. Almost all intrusive igneous rocks have crystals that are large enough to see with the naked eye, and we use the term phaneritic (from the Greek word phaneros meaning visible) to describe that. Typically that means they are larger than about 0.5 millitmeres (mm) — the thickness of a strong line made with a ballpoint pen. (If the crystals are too small to distinguish, which is typical of most volcanic rocks, we use the term aphanitic (from the Greek word aphanos - unseen) The intrusive rocks shown in Figure 3.3.5 are all phaneritic, as are those shown in Exercise 3.6.
In general, the size of crystals is proportional to the rate of cooling. The longer it takes for a body of magma to cool, the larger the crystals can grow. It is not uncommon to see an intrusive igneous rock with crystals up to 1 centimetre (cm) long. In some situations, especially toward the end of the cooling stage, the magma can become water rich. The presence of liquid water (still liquid at high temperatures because it is under pressure) promotes the relatively easy movement of ions, and this allows crystals to grow large, sometimes to several centimetres (Figure 3.4.4). Finally, as already described, if an igneous rock goes through a two-stage cooling process, its texture will be porphyritic (Figure 3.3.7).

Image Descriptions
Attributions
- Figure 3.4.1, 3.4.2, 3.4.3: © Steven Earle. CC BY.
- Figure 3.4.4: Pegmatite. Public domain.
The world's longest mountain chain found beneath the Atlantic ocean between North America, South America and Europe and Africa. The Mid-Atlantic Ridge is an example of a mid-ocean ridge, or MOR.