5.3 Deadly Hazards
Volcanic Hazards
Volcanoes are responsible for a large number of deaths, but lava is not the only danger associated with these hazards. Mount Vesuvius (Naples, Italy) is infamous for its violent explosion thousands of years ago in 79 AD when a pyroclastic flow travelled over the Roman countryside and engulfed the cities of Pompeii and Herculaneum [1]. It was not until the 18th century that we uncovered the shocking remains of these towns beneath over 10 feet of ash and the casts of people preserved within it.
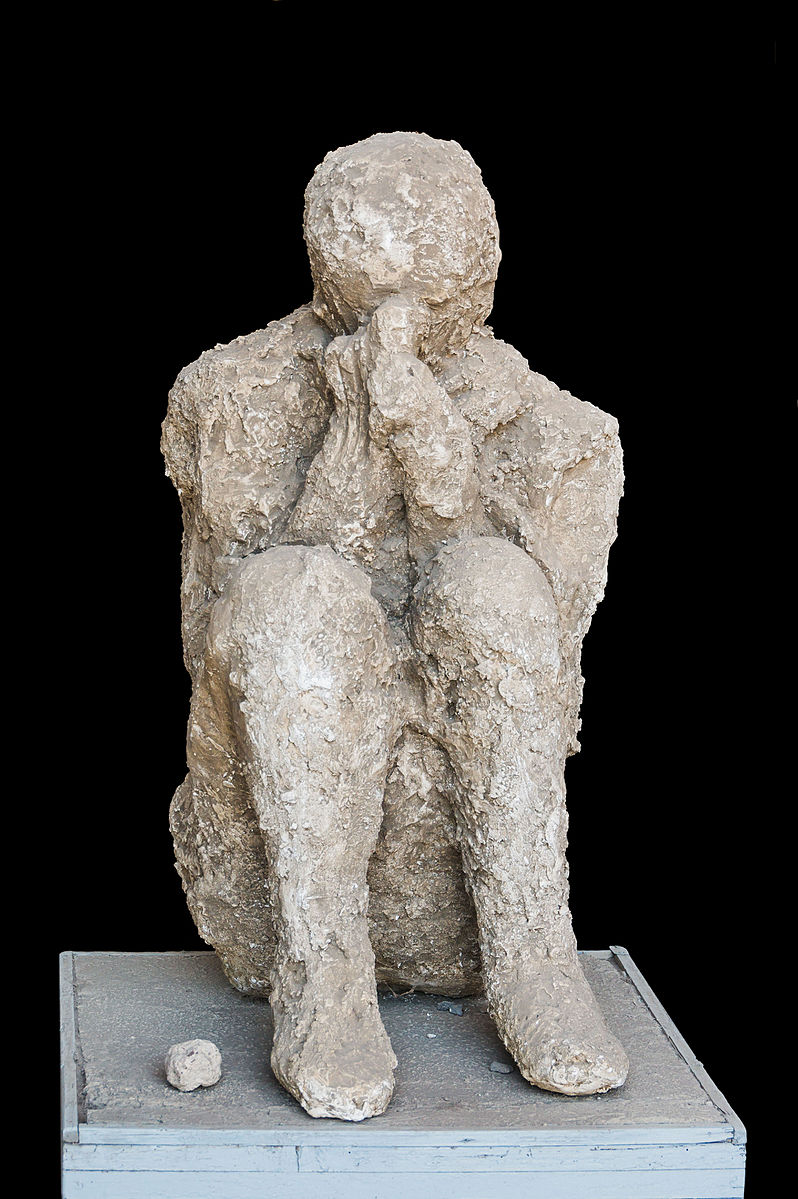
We know more about volcanic hazards over the past century because they have been better monitored and documented. We have seen enormously violent explosions, effusive lava, fast pyroclastic flows, ash, landslides, toxic gases, and more!
Pyroclastic Flows
The most dangerous type of volcanic hazard are pyroclastic flows. These flows are a mix of lava blocks, pumice, ash, and hot gases between 400 to 1,300°F! The turbulent cloud of ash and gas races down the steep flanks at high speeds at an average of 60 mph (much faster than people can run) into the valleys where farmlands grow and cities thrive [1].

Pyroclastic flows can often be expected of stratovolcanoes that contain felsic or intermediate magma. This magma is silica-rich and contains volatile gases that make it highly viscous. Therefore, these volcanoes often have very violent eruptions that are accompanied by a pyroclastic flow.
There are numerous examples of deadly pyroclastic flows. In 2014, the Mount Ontake pyroclastic flow in Japan killed 47 people. The flow was caused by magma heating groundwater into steam, which then rapidly ejected with ash and volcanic bombs. Some were killed by inhalation of toxic gases and hot ash, while volcanic bombs struck others [1]. In 1902, on the Caribbean Island Martinique, Mount Pelee erupted with a violent pyroclastic flow that destroyed the entire town of St. Pierre and killing 28,000 people in moments [3].
Lahars
How Lahars are formed:
A lahar is an Indonesian word for a mudflow that is a mixture of water, ash, rock fragments, and other debris that moves down the mountainside of a volcano (or other nearby mountains covered with freshly-erupted ash). They form from the rapid melting of snow or glaciers on volcanoes or sometimes in combination with a new eruption and heavy thunderstorm, as seen at Mt. Pinatubo.
Lahars move like a slurry of concrete, but they can move extremely fast at speeds up to 50 mph. Part of the reason they are so deadly is because they are slurry-like; they easily capture materials in their wake and they can travel very long distances like a flash flood [1].
During the 1980 Mount St. Helens eruption, lahars reached 17-miles (27 km) down the North Fork of the Toutle River. Another scenario played out when a lahar from the volcano Nevado del Ruiz Colombia, buried a town in 1985 and killed an estimated 25,000 people [1].

Tephra and Ash
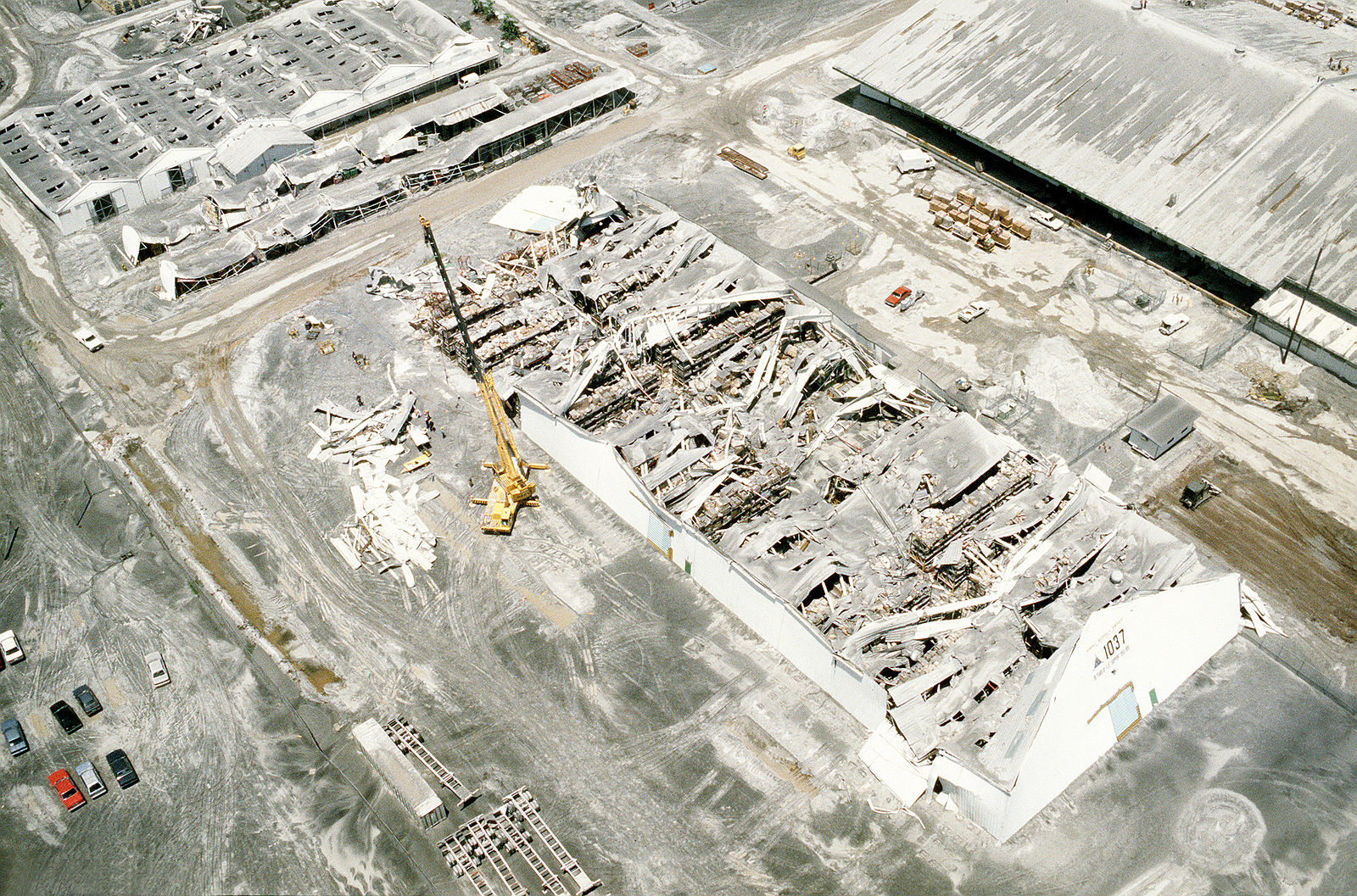
Volcanoes—mainly stratovolcanoes—eject substantial amounts of tephra and ash [1].
Tephra is heavier than ash, so it will fall closer to the volcano’s crater and vent. Large masses of tephra sometimes erupt from volcanoes and can pose deadly hazards to anyone nearby. These are called volcanic bombs [1].

Ash is much finer, but it should not be underestimated. It can be carried much longer distances away from the volcano, and that can cause more widespread issues in nearby towns and cities. A build-up of ash can collapse the roof of a building or home, and the microscopic minerals within ash will cause respiratory illnesses such as silicosis. Inhaling ash is extremely hazardous because much of it contains microscopic volcanic glass particles [4]. Imagine inhaling tiny shards of glass!
Ash will also interfere with transportation services farther away from an eruption. For example, the 2010 Eyjafjallajökull volcanic eruption in Iceland created a gigantic ash cloud that caused a significant air travel disruption in northern Europe. No one was hurt, but the cost to the world economy was estimated to be billions of dollars [3].
Volcanic Gases
Magma contains dissolved volatile gases. As magma rises toward the surface, the pressure that keeps it in

the magma chamber will start to decrease, which allows those gases to escape. Think of this process like twisting the cap of a soda bottle; the first thing to rise and escape in that initial hissing noise is gas [1]!
The types of gases that are commonly released from volcanoes include greenhouse gases such as carbon dioxide (CO2), sulfur dioxide (SO2), hydrogen sulfide (H2S), methane (CH4), and water vapor (H2O). There are also toxic and acidic gases present at volcanoes such as HF, HBr, and HCl. After gigantic volcanic explosions, some volcanic gases such as sulfur dioxide will become sulfate aerosols in the atmosphere. These aerosol particles block sunlight coming toward Earth’s surface and cause the planet to become cooler [5].
The volcanic gases can be both toxic and suffocating, and these gases sometimes are released from a volcano without an accompanying eruption. Gases were released from the Oku Volcanic Plain in Lake Nyos, Cameroon, and the carbon dioxide suffocated almost 2,000 people in 1986 [1].
***See 5.8 for Text and Media Attributions
an area on the Earth's surface where lava, ash, and/or volatile gases erupt and eventually solidify into rock.
molten rock that has erupted at the Earth's surface due to volcanic processes.
A region along Earth's lithosphere where at least two tectonic plates move apart from one another.
It’s important to classify slope failures so that we can understand what causes them and learn how to mitigate their effects. The three criteria used to describe slope failures are:
- Material: Type of material that failed (typically either bedrock or unconsolidated sediment)
- Motion: How the material moved (fall, slide, or flow).
- Rate: Speed at which the material moved.
Three types of motion associated with slope failure are:
- Fall: Material drops through the air, vertically or nearly vertically.
- Slide: Material moves as a cohesive mass along a sloping surface.
- Flow: Material moves like a fluid.
Unfortunately it’s not normally that simple. Many slope failures involve two of these types of motion, some involve all three, and in many cases, it’s not easy to tell how the material moved. The types of slope failure that we’ll cover here are summarized in Table 11.1, though there are other types of mass wasting.
[Skip Table] | |||
Failure Type | Type of Material | Type of Motion | Rate of Motion |
---|---|---|---|
Rock fall | Bedrock | Fall | Extremely rapid |
Rock slide | Bedrock | Slide | Very slow to extremely rapid |
Rock avalanche | Bedrock (slides then breaks into smaller fragments) | Flow | Extremely rapid |
Creep or solifluction | Unconsolidated materials (rock fragments, soils) | Flow or slide | Extremely slow |
Slump | Unconsolidated sediments | Slide | Very slow to moderate |
Mudflow | Unconsolidated sediments (very small silt and clay) | Flow | Moderate to Extremely rapid |
Debris flow | Unconsolidated sediments (Sand, gravel, and larger fragments) | Flow | Rapid to Extremely rapid |
Rock Fall
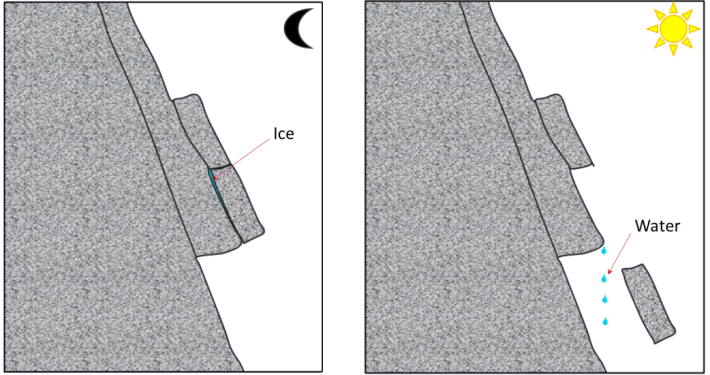
Rock fragments can break off relatively easily from steep bedrock slopes, most commonly due to frost-wedging in areas where there are many freeze-thaw cycles per year. When water freezes to form ice, its volume increases by about 8%, this causes fractures to enlarge. When the ice melts, more water can fill the larger crack, and refreeze. This process over time can cause large pieces of solid rock to fall (Fig 11.2.1). However, it can occur due to other triggers, particularly heavy rain, which adds weight or loosens material.
A rock fall in Verde Valley, Arizona, caused tons of solid material to fall to the valley below, destroying an RV but stopping short of hitting the house (Figure 11.2.2). Luckily no one was hurt in this rock fall, but living at the base of a cliff can be very dangerous and a fall is likely to happen again.

Rock Slide
A rock slide is the sliding motion of rock along a sloping surface. In most cases, the movement is parallel to a fracture, bedding, or metamorphic foliation plane, and it can range from very slow to moderately fast.
On June 23, 1925, a 38 million cubic meter rock slide occurred next to the Gros Ventre River (pronounced “grow vont”) near Jackson Hole, Wyoming. Large boulders dammed the Gros Ventre River and ran up the opposite side of the valley several hundred vertical feet. The dammed rivercreated Slide Lake, and two years later in 1927, lake levels rose high enough to destabilize the dam. The dam failed and caused a catastrophic flood that killed six people in the small downstream community of Kelly, Wyoming.

A combination of three factors caused the rock slide: 1) heavy rains and rapidly melting snow saturated the sandstone causing the underlying shale to lose its shear strength, 2) the Gros Ventre River cut through the sandstone creating an <span class="glossaryLink" style="border-bottom: 1px dotted #000000 !important;text-decoration: none !important;color: #000000 !important" aria-describedby="tt" data-cmtooltip="
">oversteepened slope, and 3) soil on top of the mountain became saturated with water due to poor drainage. The cross-section diagram shows how the parallel bedding planes between the sandstone and clay/limestone offered little friction against the slope surface as the river undercut the sandstone. Lastly, the rockslide may have been triggered by an earthquake. (3)
Rock Avalanche
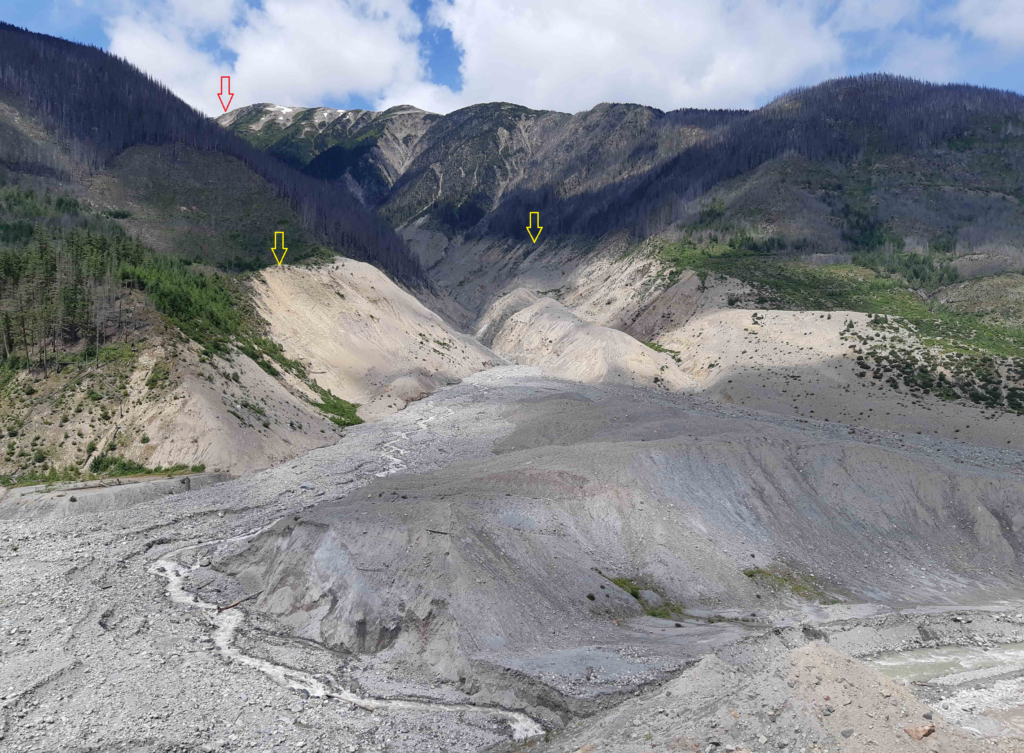
If a rock slides and then starts moving quickly (meters per second), the rock is likely to break into many small pieces, and at that point it turns into a rock avalanche in which the large and small fragments of rock move in a fluid manner supported by a cushion of air within and beneath the moving mass. The 2010 slide at Mount Meager (north of Vancouver, British Columbia, Canada) was a rock avalanche, and is the largest slope failure in Canada during historical times (Figure 11.2.5). Though no one was harmed during this event, there is a great potential for damage and loss of life from this type of mass wasting. (1)
Creep or Solifluction
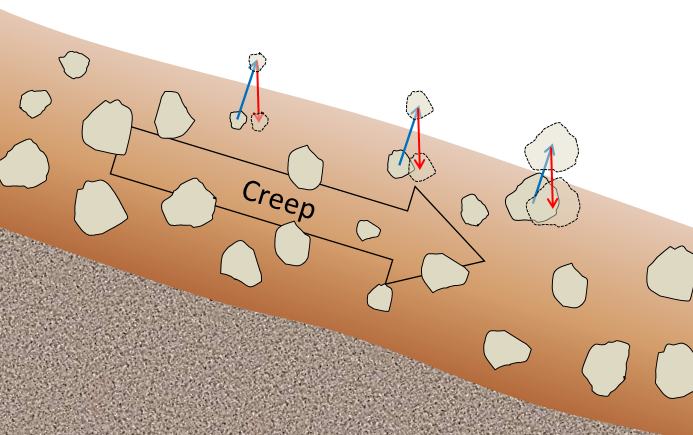
The very slow—millimeters per year to centimeters per year—movement of soil or other unconsolidated material on a slope is known as creep. Creep, which normally only affects the upper several centimeters of loose material, is typically a type of very slow flow, but in some cases, sliding may take place. Creep can be facilitated by freezing and thawing because, as shown in Figure 11.2.6, particles are lifted perpendicular to the surface by the growth of ice crystals within the soil, and then let down vertically by gravity when the ice melts. The same effect can be produced by frequent wetting and drying of the soil. In cold environments, solifluction is a more intense form of freeze-thaw-triggered creep. Creep is most noticeable on moderate-to-steep slopes where trees, fence posts, or grave markers are consistently leaning in a downhill direction. In the case of trees, they try to correct their lean by growing upright, and this leads to a curved lower trunk, shown on Figure 11.2.7.
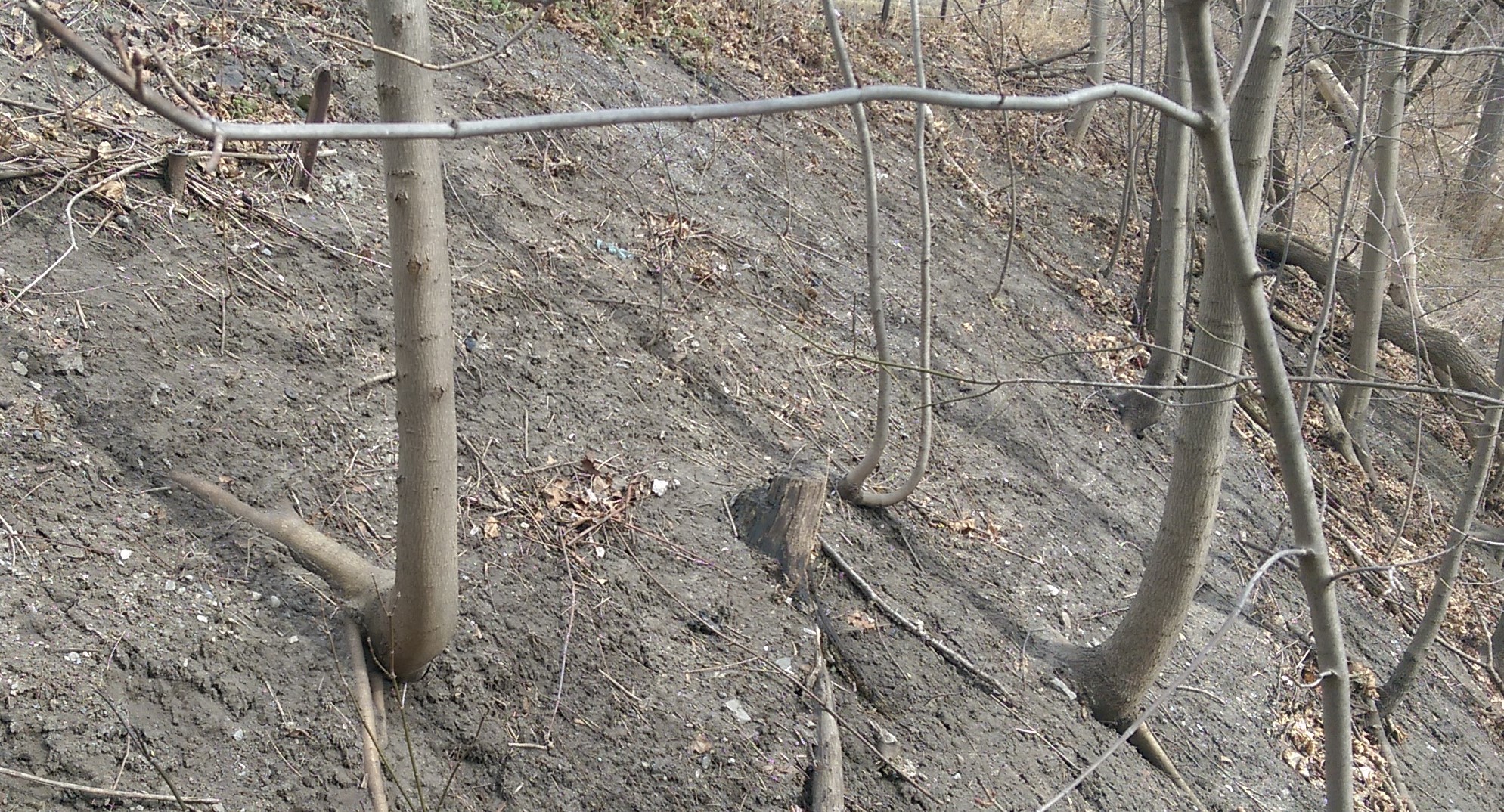
Slump
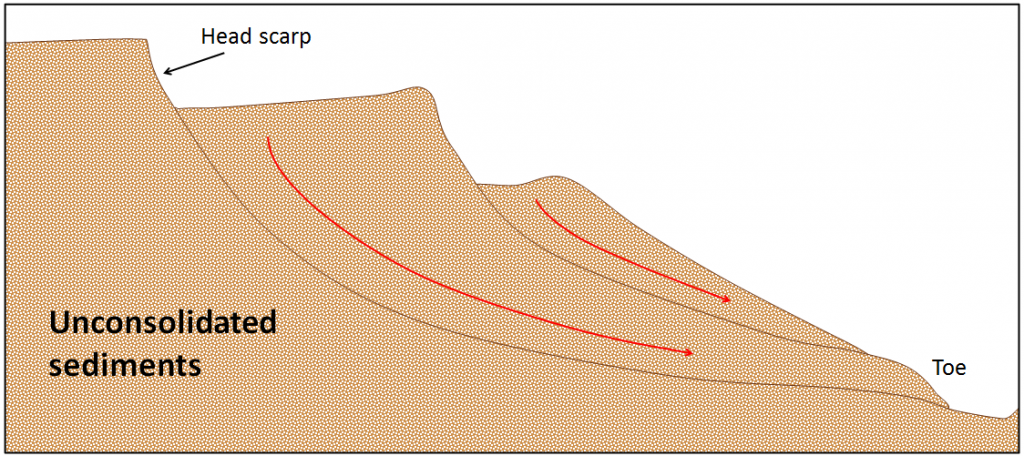
Slump is a type of slide (movement as a mass) that takes place within thick unconsolidated deposits (typically thicker than 10 meters). Slumps involve movement along one or more curved failure surfaces, with downward motion near the top and outward motion toward the bottom (Figure 11.2.8). They are typically caused by an excess of water within these materials on a steep slope.
An example of a slump in the Lethbridge area of Alberta is shown in Figure 11.2.9. This feature has likely been active for many decades, and moves a little more whenever there are heavy spring rains and significant snowmelt runoff. The toe of the slump is failing because it has been eroded by the small stream at the bottom.
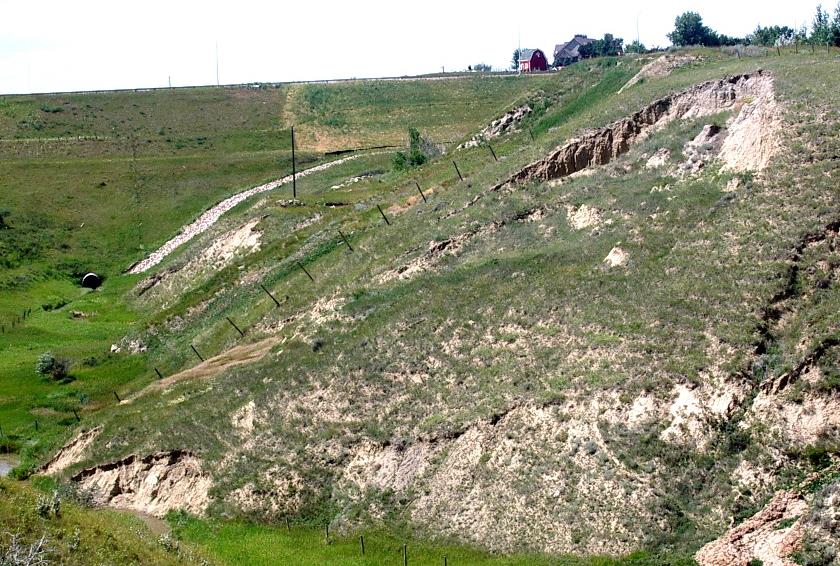
Mudflows and Debris Flows
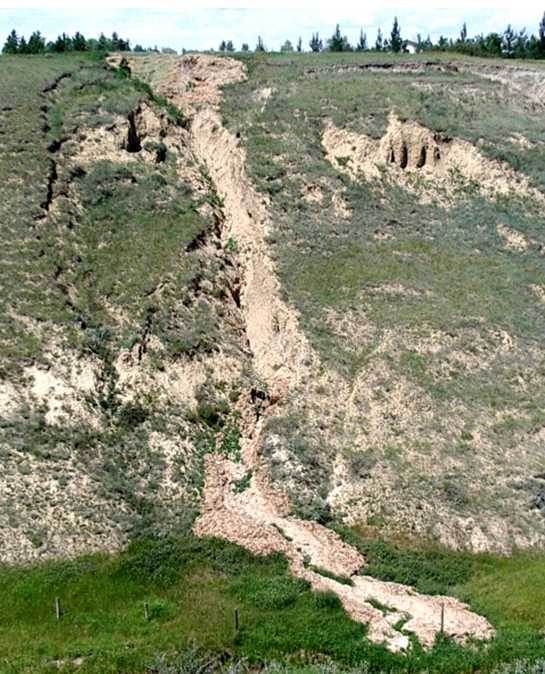
As you saw previously, when a mass of sediment becomes completely saturated with water, the mass loses strength, to the extent that the grains are pushed apart, and it will flow, even on a gentle slope. This can happen during rapid spring snowmelt or heavy rains, and is also relatively common during volcanic eruptions because of the rapid melting of snow and ice. (A mudflow or debris flow on a volcano or during a volcanic eruption is a lahar.) If the material involved is primarily sand-sized or smaller, it is known as a mudflow, such as the one shown in Figure 11.2.10.

If the material involved is gravel sized or larger, it is known as a debris flow. Because it takes more gravitational energy to move larger particles, a debris flow typically forms in an area with steeper slopes and more water than does a mudflow. In many cases, a debris flow takes place within a steep stream channel, and is triggered by the collapse of bank material into the stream. This creates a temporary dam, and then a major flow of water and debris when the dam breaks. Large amounts of debris can be carried.
volcanically ejected rock fragments or particles that are less than 2 mm.
As has already been described, igneous rocks are classified into four categories: felsic, intermediate, mafic, and ultramafic, based on either their chemistry or their mineral composition. The diagram in Figure 4.4.1 can be used to help classify igneous rocks by their mineral composition. An important feature to note on this diagram is the red line separating the non-ferromagnesian silicates in the lower left (K-feldspar, quartz, and plagioclase feldspar) from the ferromagnesian silicates in the upper right (biotite, amphibole, pyroxene, and olivine). In classifying intrusive igneous rocks, the first thing to consider is the percentage of ferromagnesian silicates. In most igneous rocks the ferromagnesian silicate minerals are clearly darker than the others, but it is still quite difficult to estimate the proportions of minerals in a rock.
Based on the position of the red line in Figure 4.4.1, it is evident that felsic rocks can have between 1% and 20% ferromagnesian silicates (the red line intersects the left side of the felsic zone 1% of the distance from the top of the diagram, and it intersects the right side of the felsic zone 20% of the distance from the top). Intermediate rocks have between 20% and 50% ferromagnesian silicates, and mafic rocks have 50% to 100% ferromagnesian silicates. To be more specific, felsic rocks typically have biotite and/or amphibole; intermediate rocks have amphibole and, in some cases, pyroxene; and mafic rocks have pyroxene and, in some cases, olivine.
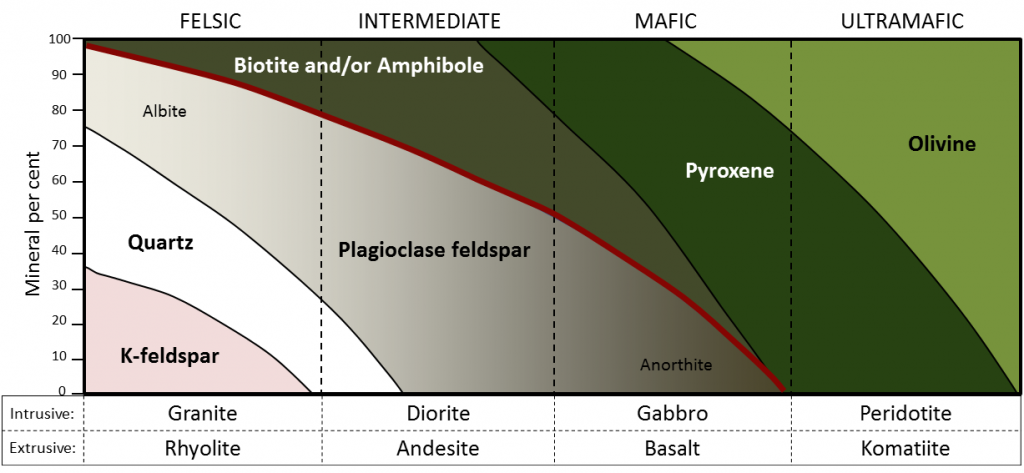
If we focus on the non-ferromagnesian silicates, it is evident that felsic rocks can have from 0% to 35% K-feldspar, from 25% to 35% quartz (the vertical thickness of the quartz field varies from 25% to 35%), and from 25% to 50% plagioclase (and that plagioclase will be sodium-rich, or albitic). Intermediate rocks can have up to 25% quartz and 50% to 75% plagioclase. Mafic rocks only have plagioclase (up to 50%), and that plagioclase will be calcium-rich, or anorthitic.
Exercise: Mineral proportions in igneous rocks

Figure 3.4.3 provides a diagrammatic representation of the proportions of dark minerals in light-coloured rocks. You can use that when trying to estimate the ferromagnesian mineral content of actual rocks, and you can get some practice doing that by completing Exercise 3.6. Be warned! Geology students almost universally over-estimate the proportion of dark minerals.

Exercise 3.6 Proportions of ferromagnesian silicates
The four igneous rocks shown below have differing proportions of ferromagnesian silicates. Estimate those proportions using the diagrams in Figure 3.4.3, and then use Figure 3.4.1 to determine the likely rock name for each one.
![]() |
![]() |
![]() |
![]() |
___% | ___% | ___% | ___% |
__________ | __________ | __________ | __________ |
See Appendix 3 for Exercise 3.6 answers.
Igneous rocks are also classified according to their textures. The textures of volcanic rocks will be discussed in Chapter 4, so here we’ll only look at the different textures of intrusive igneous rocks. Almost all intrusive igneous rocks have crystals that are large enough to see with the naked eye, and we use the term phaneritic (from the Greek word phaneros meaning visible) to describe that. Typically that means they are larger than about 0.5 millitmeres (mm) — the thickness of a strong line made with a ballpoint pen. (If the crystals are too small to distinguish, which is typical of most volcanic rocks, we use the term aphanitic (from the Greek word aphanos - unseen) The intrusive rocks shown in Figure 3.3.5 are all phaneritic, as are those shown in Exercise 3.6.
In general, the size of crystals is proportional to the rate of cooling. The longer it takes for a body of magma to cool, the larger the crystals can grow. It is not uncommon to see an intrusive igneous rock with crystals up to 1 centimetre (cm) long. In some situations, especially toward the end of the cooling stage, the magma can become water rich. The presence of liquid water (still liquid at high temperatures because it is under pressure) promotes the relatively easy movement of ions, and this allows crystals to grow large, sometimes to several centimetres (Figure 3.4.4). Finally, as already described, if an igneous rock goes through a two-stage cooling process, its texture will be porphyritic (Figure 3.3.7).

Image Descriptions
Attributions
- Figure 3.4.1, 3.4.2, 3.4.3: © Steven Earle. CC BY.
- Figure 3.4.4: Pegmatite. Public domain.
Topography of the Sea Floor
Oceans cover 71% of Earth’s surface and hold 97% of Earth’s water. The water the oceans hold is critical to plate tectonics, volcanism, and, of course, life on Earth. We know more about the surface of the Moon than the floor of the oceans. Whether this is true or not, the critical point is that the ocean floor is covered with an average of nearly 4,000 m of water, and it is pitch black below a few hundred meters, so it is not easy to discover what is down there. We know a lot more about the oceans than we used to, but there is still a great deal more to discover. (4)
Earth has had oceans for a very long time, dating back to the point where the surface had cooled enough to allow liquid water, only a few hundred million years after Earth's formation. At that time, there were no continental rocks, so the water that was here was likely spread out over the surface in one giant (but relatively shallow) ocean. (4)
We examined the seafloor's topography from the perspective of plate tectonics, but here we are going to take another look at the essential features from an oceanographic perspective. The essential features are the extensive continental shelves less than 250 m deep (pink); the vast deep abyssal plains between 3,000 and 6,000 m deep (light and dark blue); the mid-Atlantic ridge, in many areas shallower than 3,000 m; and the deep ocean trench north of Puerto Rico. (4) These features are connected by continental slopes, which is the transition area between continental shelves and abyssal planes.
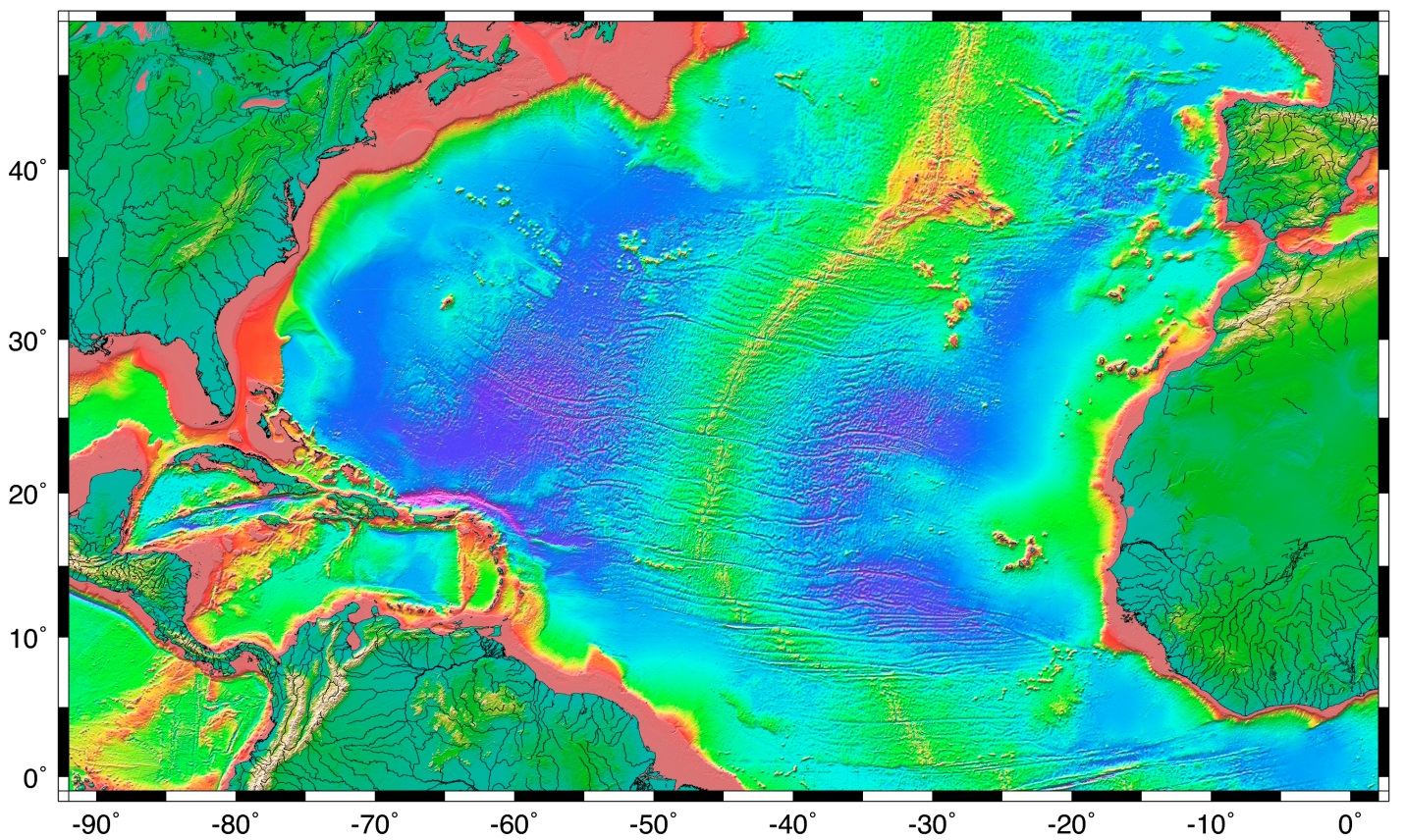
Of course, it is more complicated than this, even in this simplified form. Figure 8.4.2 shows a generalized cross-section of the Pacific Ocean which has short continental shelves that quickly turn to continental slopes, dropping from about 200 m to several thousand meters over a distance of a few hundred kilometers. The continental slopes connect to abyssal plains – exceedingly flat and from 4,000 m to 6,000 m deep; volcanic seamounts and islands; and trenches at subduction zones that are up to 11,000 m deep.
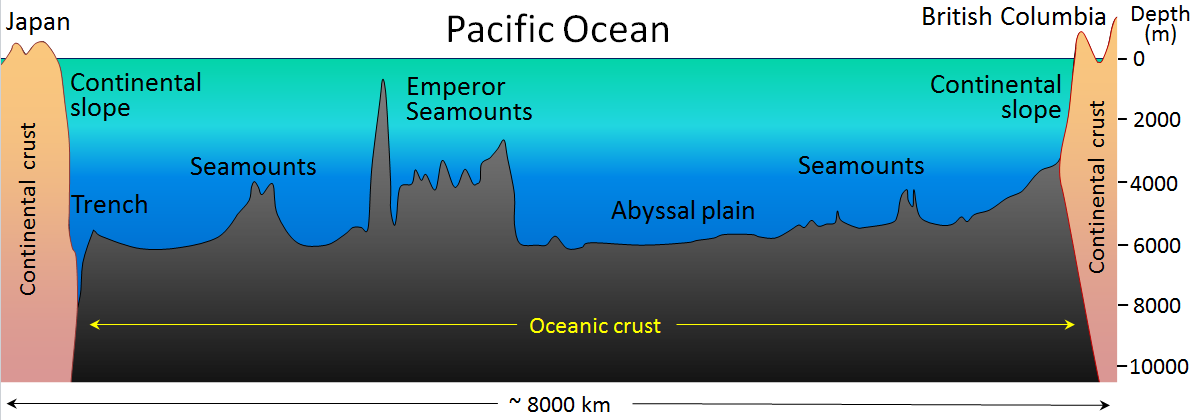
The ocean floor is entirely underlain by mafic oceanic crust, while the continental slopes are underlain by felsic continental crust (mostly granitic and sedimentary rocks). Moreover, the denser oceanic crust floats lower on the mantle than continental crust does, and that is why oceans are oceans. Although the temperature of the ocean surface varies widely, from a few degrees either side of freezing in polar regions to over 25°C in the tropics, in most parts of the ocean, the water temperature is around 10°C at 1,000 m depth and about 4°C from 2,000 m depth to the bottom. (4)
The deepest parts of the ocean are within the subduction trenches, and the deepest of these is the Marianas Trench in the southwestern Pacific (near Guam) at 11,000 m. Other trenches in the southwestern Pacific are over 10,000 m deep; the Japan Trench is over 9,000 m deep, and the Puerto Rico and Chile-Peru Trenches are over 8,000 m deep. Shallow trenches tend to be that way because they have significant sediment infill. There is no recognizable trench along the subduction zone of the Juan de Fuca Plate because it has been filled with sediments from the Fraser and Columbia Rivers. (4)
Landforms of Coastal Erosion
Large waves crashing onto a shore bring a tremendous amount of energy that has a significant eroding effect, and several unique erosion features commonly form on rocky shores with strong waves. When waves approach an irregular shore, they are slowed down to varying degrees, depending on differences in the water depth, and as they slow, they are bent or refracted. That energy is evenly spaced out in the deep water, but because of refraction, the waves' energy, which moves perpendicular to the wave crests, is being focused on the headlands. On irregular coasts, the headlands receive much more wave energy than the intervening bays, and thus they are more strongly eroded. The result of this is coastal straightening. An irregular coast, like the west coast of Vancouver Island, will eventually become straightened, although that process will take millions of years. (4)
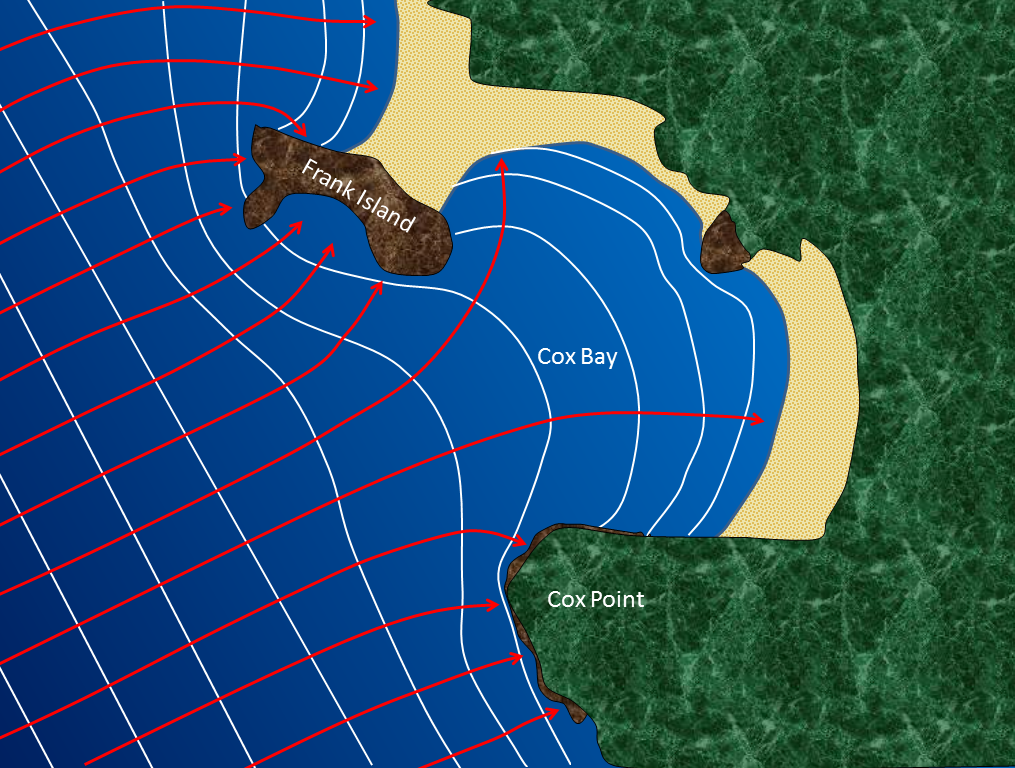
Wave erosion is highest in the surf zone, where the wave base is impinging strongly on the seafloor and where the waves are breaking. The result is that the substrate in the surf zone is typically eroded to a flat surface known as a wave-cut platform. A wave-cut platform extends across the intertidal zone. (4)
Resistant rock that does not get eroded entirely during the formation of a wave-cut platform will remain behind to form a stack. Here the different layers of the sedimentary rock have different resistance to erosion. The upper part of this stack is made up of rock that resisted erosion, and that rock has protected a small pedestal of underlying softer rock. The softer rock will eventually be eroded, and the big rock will become just another boulder on the beach.
Arches and sea caves are related to stacks because they all form because of the erosion of non-resistant rock. (4)

Submarine Canyons
Submarine canyons are narrow and deep canyons located in the marine environment on continental shelves. They typically form at the mouths of sizeable landward river systems, both by cutting down into the continental shelf during low sea levels and by continual material slumping or flowing down from the mouth of the river or a delta. Underwater currents rich in sediment pass through the canyons, erode them and drain onto the ocean floor. Steep delta faces and underwater flows of sediments are released down the continental slope as underwater landslides, called turbidity flows. The erosive action of this type of flow continues to cut the canyon, and eventually, fan-shaped deposits develop on the ocean floor beyond the continental slope. (4)

Landforms of Coastal Deposition
Some coastal areas are dominated by erosion, an example being the Pacific coast of Canada and the United States, while others are dominated by deposition, examples being the Atlantic and Caribbean coasts of the United States. However, on almost all coasts, deposition and erosion are happening to vary degrees most of the time, although in various places. On deposition-dominant coasts, the coastal sediments are still being eroded from some areas and deposited in others.
The main factor in determining if the coast is dominated by erosion or deposition is its history of tectonic activity. A coast like that of British Columbia is tectonically active, and compression and uplift have been going on for tens of millions of years. This coast has also been uplifted during the past 15,000 years by isostatic rebound due to deglaciation. The coasts of the United States along the Atlantic and the Gulf of Mexico have not seen significant tectonic activity in a few hundred million years, and except in the northeast, have not experienced post-glacial uplift. These areas have relatively little topographic relief, and there is now minimal erosion of coastal bedrock. (4)
On coasts dominated by depositional processes, most of the sediment being deposited typically comes from large rivers. An obvious example is where the Mississippi River flows into the Gulf of Mexico at New Orleans; another is the Fraser River in Vancouver. No large rivers bring sandy sediments to the west coast of Vancouver Island, but there are still long and wide sandy beaches there. In this area, most of the sand comes from glaciofluvial sand deposits situated along the shore behind the beach, and some come from the erosion of the rocks on the headlands. (4)
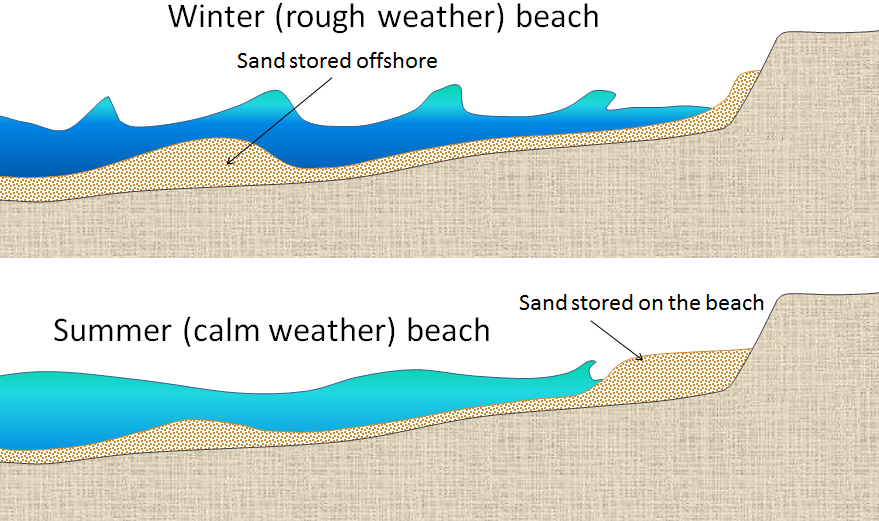
Most beaches go through a seasonal cycle because conditions change from summer to winter. In summer, sea conditions are calm with long-wavelength, low-amplitude waves generated by distant winds. Winter conditions are rougher, with shorter-wavelength, higher-amplitude waves caused by strong local winds. As seen in Figure 14.4.7, the heavy seas of winter gradually erode sand from beaches, moving it to an underwater sandbar offshore. The gentler waves of summer gradually push this sand back toward the shore, creating a broader and flatter beach. (4)
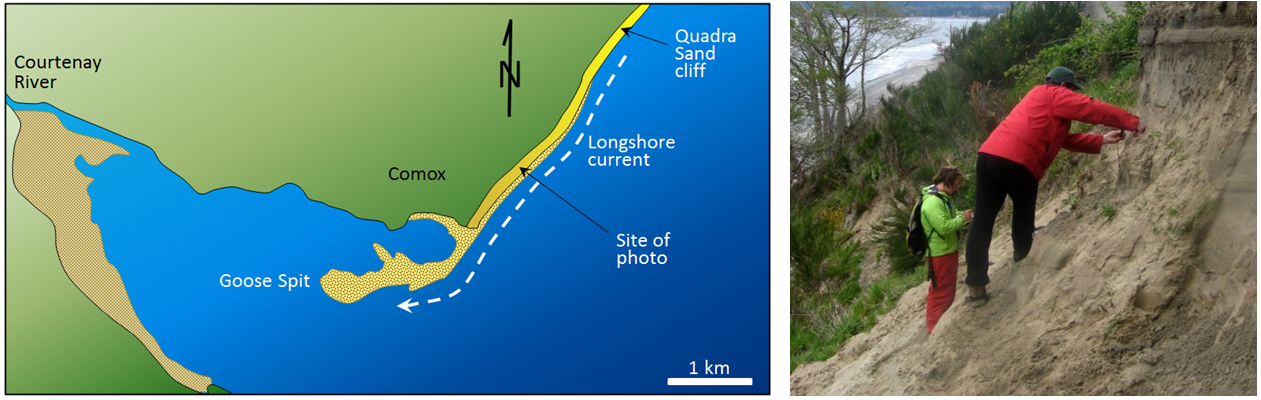
The evolution of sandy depositional features on seacoasts is primarily influenced by waves and currents, especially longshore currents. As sediment is transported along a shore, either it is deposited on beaches, or it creates other depositional features. For example, a spit is an elongated sandy deposit that extends out into open water in the direction of a longshore current. (4)
A spit that extends across a bay to the extent of closing, or almost closing it off, is known as a baymouth bar. Most bays have streams flowing into them, and since this water must get out, rarely, a baymouth bar will completely close the entrance to a bay. In areas where there is sufficient sediment being transported, and there are nearshore islands, a tombolo may form.
Tombolos are common where islands are abundant, and they typically form where there is a wave shadow behind a nearshore island. This becomes an area with reduced energy, and so the longshore current slows, and sediments accumulate. Eventually, enough sediments accumulate to connect the island to the mainland with a tombolo. (4)

In areas where coastal sediments are abundant and coastal relief is low (because there has been little or no recent coastal uplift), it is common for barrier islands to form. Barrier islands are elongated islands composed of sand that form a few kilometers away from the mainland. They are common along the US Gulf Coast from Texas to Florida, and along the US Atlantic Coast from Florida to Massachusetts. North of Boston, the coast becomes rocky, partly because that area has been affected by a post-glacial crustal rebound. (4) Barrier islands do an excellent job of blocking incoming storm surges from hurricanes, but since they are made almost entirely of sand they shift and move with every storm event. Though they are largely unstable for building and infrastructure, many people live on barrier islands and have to regularly face coastal hazards. The 2000 U.S. Census estimates that there are 1.4 million people living on barrier islands, with a population density of three times that of the coast.
originating from a feldspar and silica-rich magma/lava composition.
Scientific Method in Geosciences: Multiple hypotheses, Multiple modes of Inquiry
How is science made in Earth Sciences? Why should you care? Even if you are not interested in becoming an Earth scientist, or any kind of scientist, understanding how science works is a source of empowerment. This is because science and scientific thinking are at the heart of our modern way of life and they influence every aspect of our lives. Understanding how science works can help you discern fact from fiction and inform your life choices and political decisions.
Modern science is based on the scientific method, the idea in science that phenomena and ideas need to be scrutinized using hypothesizing, experimentation, and analysis. This can eventually result in a consensus or scientific theory.
The scientific method, is a procedure that follows these steps:
- Formulate a question or observe a problem
- Apply objective experimentation and observation
- Analyze collected data and Interpret results.
- Devise an evidence-based theory.
- Submit findings to peer review.
Let's see how scientific inquiry works in Earth science.
1. Observation, problem, or research question
The procedure begins when scientists identify a problem or research question, such as a geological phenomenon that is not well explained in the scientific community’s collective knowledge. This step usually involves reviewing the scientific literature to establish what is known and to consult previous studies related to the question.
Earth scientists can study processes they cannot observe directly, because they happen over long periods of time, or happened long ago, or happen in a remote location. An example of the last one would be the Earth's core. To circumvent these challenges, Earth scientists have developed strategies to test their hypothesis, these strategies make up the scientific method of geosciences.
2. Hypothesis
Once the scientists define the problem or question, they propose a possible answer, a hypothesis
hypotheses to gather data from multiple lines. To test hypotheses, scientists use methods drawn from other sciences such as chemistry, physics, biology, or even engineering.
3. Testing Hypotheses: Experiments and Revisions

The next step is developing an <span aria-describedby="tt" data-cmtooltip="
Earth scientists conduct classic experiments in the lab, however, an
An experiment can take other forms such as:
- Observing natural processes and their products in the field and comparing them to those found in the rock record. E.g., A sedimentologist studies how wind moves and forms dunes and different ripples in a desert. This knowledge helps her to interpret ripple structures found in rocks, and even to interpret dunes and aeolian processes from images collected on Mars!
- Studying changes across time or space. E.g., an atmospheric scientist analyzes how the composition of the atmosphere has changed since we started measuring it.
- Using physical models. This is more akin to the "classic experiment". E.g., a team of scientists build a model for landslides using a long and steep ramp and flushing down different materials, and changing the ramp angle.
- Using computer models. E.g., climatologists develop computational models to study the climate system and make predictions. These scientific models undergo rigorous scrutiny and testing by collaborating and competing groups of scientists around the world.
- Considering multiple lines of evidence. To establish a scientific finding, all lines of evidence must converge, that means that all the results you collect using different methods must agree with the finding, the math must be sound, and the methods must be thoroughly described.
Regardless of what form an <span aria-describedby="tt" data-cmtooltip="
">experiment takes, it always includes the systematic gathering of <span aria-describedby="tt" data-cmtooltip="
">objective data. The scientists interpret this data to determine whether it contradicts or supports the <span aria-describedby="tt" data-cmtooltip="
">hypothesis (step 2). If the results contradict the hypothesis, then scientists can revise it and test it again. When a <span aria-describedby="tt" data-cmtooltip="
">hypothesis holds up under experimentation, it is ready to be shared with other experts in the field. The findings are scrutinized by the scientific community through the process of peer review.
Backyard Geology: Arizona Wildfire Study in Saguaro National Park
Arizona ranks high in the number of individual fires, as well as acres burned, every year. Below are the top 10 states for wildfires:
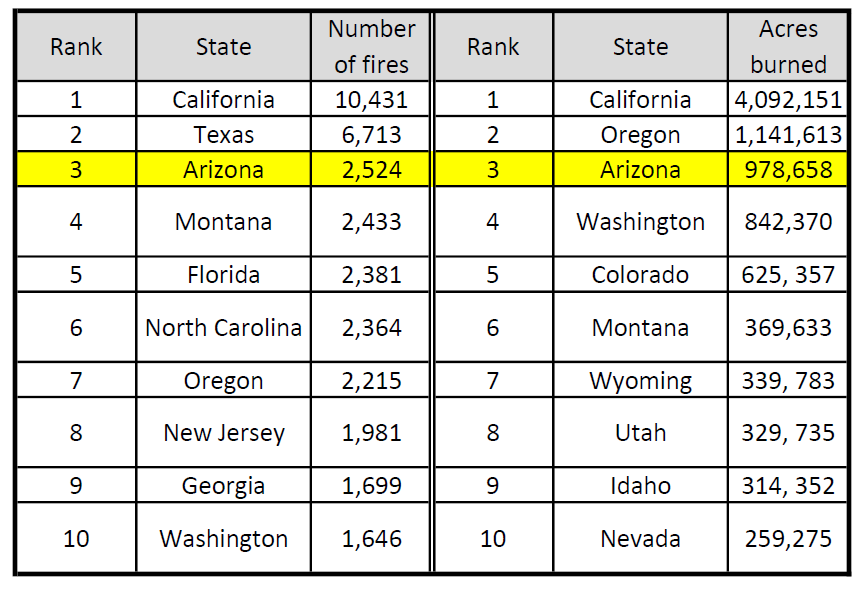
In 2020, Arizona ranked 3rd out of all states in both categories, with 2,524 individual fires and 978,568 acres burned. Why does Arizona have so many fires? Wildfire risk depends on many factors, including temperature, soil moisture, and the presence of vegetation (fuel) to burn. Certainly the dry, hot climate plays a role, and climate change intensifies drought conditions, but what contributes to the fuel available to burn?
It was determined in a previous study that invasive buffelgrass is fire-adapted, meaning it establishes, persists, and spreads following fire. It also outcompetes native vegetation for resources, supplies material that can be readily burned, and connects areas subjected to wildfires. Therefore, can we control or remove buffelgrass in the Arizona wilderness?
- Research Question: Invasive buffelgrass contributes to wildfires, can it be controlled or removed in wilderness areas?
- Hypothesis: If buffelgrass can be controlled, the intensity and interconnectedness of wildfires will lessen and cause less devastation.
- Experiment: Herbicides were used to control dense patches of buffelgrass in remote areas. The herbicide was applied with the use of helicopters.
- Results:
4. Peer review, publication, and replication
Science is a social process. Scientists share the results of their research in conferences and by publishing articles in scientific journals, such as Science and Nature. Reputable journals and academic outlets will not publish an experimental study until they have determined its methods are scientifically rigorous and the evidence supports the conclusions. Before they publish the article, scientific experts in the field scrutinize the methods, results, and discussion; the peer review process. Once an article is published, other scientists may attempt to replicate the results. This replication is necessary to confirm the reliability of the study’s reported results. A <span aria-describedby="tt" data-cmtooltip="
">hypothesis that seemed compelling in one study might be proven false in studies conducted by other scientists. New technology can be applied to published studies, which can aid in confirming or rejecting once-accepted ideas and/or <span aria-describedby="tt" data-cmtooltip="
">hypotheses.
5. Theory development

In casual conversation, the word <span aria-describedby="tt" data-cmtooltip="
">theory implies guesswork or speculation. In the language of science, an explanation or conclusion made into a <span aria-describedby="tt" data-cmtooltip="
">theory carries much more weight because experiments support it and the scientific community widely accepts it. A <span aria-describedby="tt" data-cmtooltip="
">hypothesis that has been repeatedly confirmed through documented and independent studies eventually becomes accepted as a scientific <span aria-describedby="tt" data-cmtooltip="
">theory.
While a <span aria-describedby="tt" data-cmtooltip="
">hypothesis provides a tentative explanation before an <span aria-describedby="tt" data-cmtooltip="
">experiment, a <span aria-describedby="tt" data-cmtooltip="
">theory is the best explanation after being confirmed by multiple independent experiments. Confirmation of a <span aria-describedby="tt" data-cmtooltip="
">theory may take years, or even longer. For example, the scientific community initially dismissed the <span aria-describedby="tt" data-cmtooltip="
">continental drift <span aria-describedby="tt" data-cmtooltip="
">hypothesis first proposed by Alfred Wegener in 1912 (see Chapter 2). After decades of additional evidence collection by other scientists using more advanced technology, Wegener’s <span aria-describedby="tt" data-cmtooltip="
">hypothesis was accepted and revised as the <span aria-describedby="tt" data-cmtooltip="
">theory of <span aria-describedby="tt" data-cmtooltip="
The <span aria-describedby="tt" data-cmtooltip="
">theory of evolution by natural selection is another example. Originating from the work of Charles Darwin in the mid-19th century, the <span aria-describedby="tt" data-cmtooltip="
">theory of evolution has withstood generations of scientific testing for falsifiability. It has been updated and revised to accommodate knowledge gained by using modern technologies, but the latest evidence continues to support the <span aria-describedby="tt" data-cmtooltip="
">theory of evolution.
Science
We have covered the scientific method of the geosciences. However, science is a human endeavor. For generations, Indigenous peoples have accrued empirical knowledge of the natural world, including the lithosphere. Greg Cajete uses the name Native Science to refer to "the collective heritage of human experience with the natural world" (Cajete, 2000). Geologic expertise served tribal peoples in many of the same ways that Western geology serves modern civilizations. For example, Native Americans in Cascadia record volcanic events in stories, this transmits cross-generational awareness of volcanic hazards; the Muiscas in Colombia knew how to find, mine and process the gold to make beautiful art pieces; and the Puebloans of the North American Southwest managing limited water resources using an impressive net of canals that we still use for our benefit. Western and Native scientists can collaborate to further our understanding of the Earth systems and to remember how to live well on our planet.
Science is a dynamic process. Technological advances, breakthroughs in interpretation, and new observations continuously refine our understanding of Earth. We will never stop learning about our Earth. As new findings are published, we must revise and update our scientific knowledge and discard ideas that are proven false by new observations. Science is a living entity.
In conclusion, Earth scientists do not use a single, all-encompassing "scientific method". Instead, multiple modes of inquiry respond to the complexity and spatial and temporal scales of Earth systems.
"The unique thing about the geosciences is that the knowledge, skills, and methods are brought together, refined, and evolved over time to make them most suitable for understanding the complex processes of Earth, its working in the past and the present, and its likely behavior in the future." (Manduca and Kastens, 2012).
Key Takeaways
- Earth scientists work on challenging problems that face humanity on topics such as climate change, human impacts on Earth, and hazards to humans.
- Besides the classical laboratory experiment, Earth scientists construct models or use indirect methods to study the Earth.
- Scientific results are not valid or useful unless other scientists can reproduce them. Research results undergo scrutiny by the community before and after being published.
- The study of geological and environmental issues requires multiple disciplines and the interplay of multiple methods.
- Scientific thinking advances through collaboration and community.
GeoEthics

Geoscientists must act in ethical ways to contribute to the welfare of human beings. The saying "with great knowledge comes great responsibility" holds true for Earth and environmental scientists. Earth scientist must uphold high standards in research and conduct. The research and reflection upon the values which underpin behaviors and practices between humans and Earth systems is the arena of the "Geoethics" (Di Capua and Peppoloni, 2019). The International Association for Promoting Geoethics (IAPG) provide tools to "understand the complex relationship between human action on ecosystems and the decisions geoscientists make in the discipline that impact society, including improving the awareness of professionals, students, decision-makers, media operators, and the public on an accountable and ecologically sustainable development." Source: https://www.geoethics.org/geoethics-school
All of us, human beings, have responsibilities to Earth. We do not exist apart from this planet and our behaviors impact other people, other species, the larger biosphere, hydrosphere, atmosphere, and lithosphere. Our actions impact the Earth system. As you progress in your learning, strive to think critically and to identify ethical issues. Do not be afraid to question and discuss your observations with your instructor and with peers. Just remember to do so respectfully, considering other points of view and practicing active listening.
molten rock that can be found beneath the Earth's surface.
Time is the dimension that sets geology apart from most other sciences. Geological time is vast, and Earth has changed enough over that time that some of the rock types that formed in the past could not form today. Furthermore, as we’ve discussed, even though most geological processes are very, very slow, the vast amount of time that has passed has allowed for the formation of extraordinary geological features, as shown in Figure 8.0.1.

We have numerous ways of measuring geological time. We can tell the relative ages of rocks (for example, whether one rock is older than another) based on their spatial relationships; we can use fossils to date sedimentary rocks because we have a detailed record of the evolution of life on Earth; and we can use a range of isotopic techniques to determine the actual ages (in millions of years) of igneous and metamorphic rocks.
But just because we can measure geological time doesn’t mean that we understand it. One of the biggest hurdles faced by geology students—and geologists as well—in mastering geology, is to really come to grips with the slow rates at which geological processes happen and the vast amount of time involved.\
Learning Objectives
After carefully reading this chapter, completing the exercises within it, and answering the questions at the end, you should be able to:
- Apply basic geological principles to the determination of the relative ages of rocks.
- Explain the difference between relative and absolute age-dating techniques.
- Summarize the history of the geological time scale and the relationships between eons, eras, periods, and epochs.
- Understand the importance and significance of unconformities.
- Estimate the age of a rock based on the fossils that it contains.
- Describe some applications and limitations of isotopic techniques for geological dating.
- Use isotopic data to estimate the age of a rock.
- Describe the techniques for dating geological materials using tree rings and magnetic data.
- Explain why an understanding of geological time is critical to both geologists and the public in general.
Media Attributions
- © Steven Earle. CC BY.
Gases dissolved within magma or lava.
As previously discussed in section 3.9, a rock is a collection of consolidated minerals. Rocks are grouped into three main categories based on how they form:
- Igneous: formed from the cooling and crystallization of molten rock.
- Sedimentary: formed when weathered fragments of other rocks are buried, compressed, and cemented together, or when minerals precipitate directly from solution
- Metamorphic: formed by alteration (due to heat, pressure, and/or chemical action) of a pre-existing igneous or sedimentary rock
The focus of this chapter is on Igneous rocks, though it is important to remember this is just a piece of the rock cycle.
Learning Objectives
After carefully reading this chapter, completing the exercises within it, and answering the questions at the end, you should be able to:
- Describe the rock cycle and the types of processes that lead to the formation of igneous, sedimentary, and metamorphic rocks, and explain why there is an active rock cycle on Earth.
- Explain the concept of partial melting and describe the geological processes that lead to melting.
- Describe, in general terms, the range of chemical compositions of magmas.
- Discuss the processes that take place during the cooling and crystallization of magma, and the typical order of crystallization according to the Bowen reaction series.
- Explain how magma composition can be changed by fractional crystallization and partial melting of the surrounding rocks.
- Apply the criteria for igneous rock classification based on mineral proportions.
- Describe the origins of phaneritic, porphyritic, and pegmatitic rock textures.
- Identify plutons on the basis of their morphology and their relationships to the surrounding rocks.
- Explain the origin of a chilled margin.
As previously discussed in section 3.9, a rock is a collection of consolidated minerals. Rocks are grouped into three main categories based on how they form:
- Igneous: formed from the cooling and crystallization of molten rock.
- Sedimentary: formed when weathered fragments of other rocks are buried, compressed, and cemented together, or when minerals precipitate directly from solution
- Metamorphic: formed by alteration (due to heat, pressure, and/or chemical action) of a pre-existing igneous or sedimentary rock
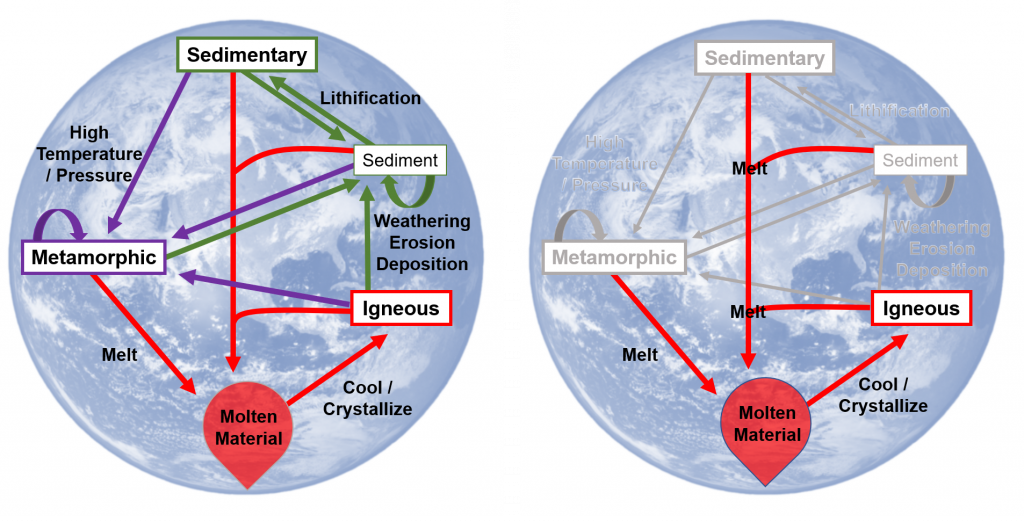
The focus of this chapter is on Igneous rocks, though it is important to remember this is just a piece of the rock cycle.
Learning Objectives
After carefully reading this chapter, completing the exercises within it, and answering the questions at the end, you should be able to:
- Describe the rock cycle and the types of processes that lead to the formation of igneous, sedimentary, and metamorphic rocks, and explain why there is an active rock cycle on Earth.
- Explain the concept of partial melting and describe the geological processes that lead to melting.
- Describe, in general terms, the range of chemical compositions of magmas.
- Discuss the processes that take place during the cooling and crystallization of magma, and the typical order of crystallization according to the Bowen reaction series.
- Explain how magma composition can be changed by fractional crystallization and partial melting of the surrounding rocks.
- Apply the criteria for igneous rock classification based on mineral proportions.
- Describe the origins of phaneritic, porphyritic, and pegmatitic rock textures.
- Identify plutons on the basis of their morphology and their relationships to the surrounding rocks.
- Explain the origin of a chilled margin.
ejected rock and particle fragments from volcanic eruptions.
As has already been described, igneous rocks are classified into four categories: felsic, intermediate, mafic, and ultramafic, based on either their chemistry or their mineral composition. The diagram in Figure 4.4.1 can be used to help classify igneous rocks by their mineral composition. An important feature to note on this diagram is the red line separating the non-ferromagnesian silicates in the lower left (K-feldspar, quartz, and plagioclase feldspar) from the ferromagnesian silicates in the upper right (biotite, amphibole, pyroxene, and olivine). In classifying intrusive igneous rocks, the first thing to consider is the percentage of ferromagnesian silicates. In most igneous rocks the ferromagnesian silicate minerals are clearly darker than the others, but it is still quite difficult to estimate the proportions of minerals in a rock.
Based on the position of the red line in Figure 4.4.1, it is evident that felsic rocks can have between 1% and 20% ferromagnesian silicates (the red line intersects the left side of the felsic zone 1% of the distance from the top of the diagram, and it intersects the right side of the felsic zone 20% of the distance from the top). Intermediate rocks have between 20% and 50% ferromagnesian silicates, and mafic rocks have 50% to 100% ferromagnesian silicates. To be more specific, felsic rocks typically have biotite and/or amphibole; intermediate rocks have amphibole and, in some cases, pyroxene; and mafic rocks have pyroxene and, in some cases, olivine.
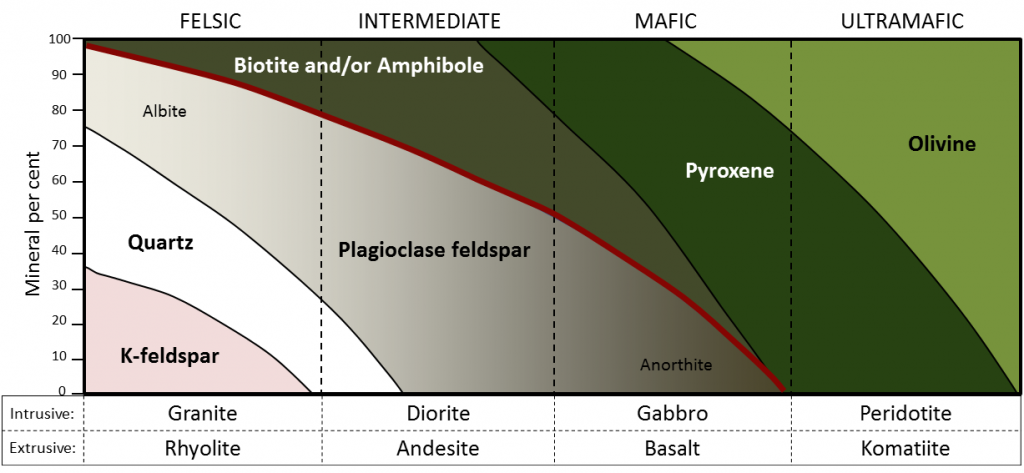
If we focus on the non-ferromagnesian silicates, it is evident that felsic rocks can have from 0% to 35% K-feldspar, from 25% to 35% quartz (the vertical thickness of the quartz field varies from 25% to 35%), and from 25% to 50% plagioclase (and that plagioclase will be sodium-rich, or albitic). Intermediate rocks can have up to 25% quartz and 50% to 75% plagioclase. Mafic rocks only have plagioclase (up to 50%), and that plagioclase will be calcium-rich, or anorthitic.
Exercise: Mineral proportions in igneous rocks

Figure 3.4.3 provides a diagrammatic representation of the proportions of dark minerals in light-coloured rocks. You can use that when trying to estimate the ferromagnesian mineral content of actual rocks, and you can get some practice doing that by completing Exercise 3.6. Be warned! Geology students almost universally over-estimate the proportion of dark minerals.

Exercise 3.6 Proportions of ferromagnesian silicates
The four igneous rocks shown below have differing proportions of ferromagnesian silicates. Estimate those proportions using the diagrams in Figure 3.4.3, and then use Figure 3.4.1 to determine the likely rock name for each one.
![]() |
![]() |
![]() |
![]() |
___% | ___% | ___% | ___% |
__________ | __________ | __________ | __________ |
See Appendix 3 for Exercise 3.6 answers.
Igneous rocks are also classified according to their textures. The textures of volcanic rocks will be discussed in Chapter 4, so here we’ll only look at the different textures of intrusive igneous rocks. Almost all intrusive igneous rocks have crystals that are large enough to see with the naked eye, and we use the term phaneritic (from the Greek word phaneros meaning visible) to describe that. Typically that means they are larger than about 0.5 millitmeres (mm) — the thickness of a strong line made with a ballpoint pen. (If the crystals are too small to distinguish, which is typical of most volcanic rocks, we use the term aphanitic (from the Greek word aphanos - unseen) The intrusive rocks shown in Figure 3.3.5 are all phaneritic, as are those shown in Exercise 3.6.
In general, the size of crystals is proportional to the rate of cooling. The longer it takes for a body of magma to cool, the larger the crystals can grow. It is not uncommon to see an intrusive igneous rock with crystals up to 1 centimetre (cm) long. In some situations, especially toward the end of the cooling stage, the magma can become water rich. The presence of liquid water (still liquid at high temperatures because it is under pressure) promotes the relatively easy movement of ions, and this allows crystals to grow large, sometimes to several centimetres (Figure 3.4.4). Finally, as already described, if an igneous rock goes through a two-stage cooling process, its texture will be porphyritic (Figure 3.3.7).

Image Descriptions
Attributions
- Figure 3.4.1, 3.4.2, 3.4.3: © Steven Earle. CC BY.
- Figure 3.4.4: Pegmatite. Public domain.
As has already been described, igneous rocks are classified into four categories: felsic, intermediate, mafic, and ultramafic, based on either their chemistry or their mineral composition. The diagram in Figure 4.4.1 can be used to help classify igneous rocks by their mineral composition. An important feature to note on this diagram is the red line separating the non-ferromagnesian silicates in the lower left (K-feldspar, quartz, and plagioclase feldspar) from the ferromagnesian silicates in the upper right (biotite, amphibole, pyroxene, and olivine). In classifying intrusive igneous rocks, the first thing to consider is the percentage of ferromagnesian silicates. In most igneous rocks the ferromagnesian silicate minerals are clearly darker than the others, but it is still quite difficult to estimate the proportions of minerals in a rock.
Based on the position of the red line in Figure 4.4.1, it is evident that felsic rocks can have between 1% and 20% ferromagnesian silicates (the red line intersects the left side of the felsic zone 1% of the distance from the top of the diagram, and it intersects the right side of the felsic zone 20% of the distance from the top). Intermediate rocks have between 20% and 50% ferromagnesian silicates, and mafic rocks have 50% to 100% ferromagnesian silicates. To be more specific, felsic rocks typically have biotite and/or amphibole; intermediate rocks have amphibole and, in some cases, pyroxene; and mafic rocks have pyroxene and, in some cases, olivine.
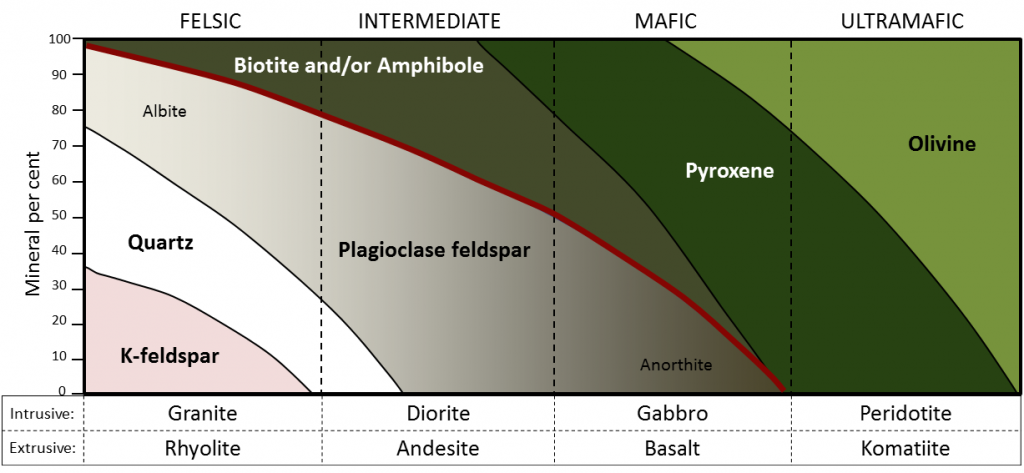
If we focus on the non-ferromagnesian silicates, it is evident that felsic rocks can have from 0% to 35% K-feldspar, from 25% to 35% quartz (the vertical thickness of the quartz field varies from 25% to 35%), and from 25% to 50% plagioclase (and that plagioclase will be sodium-rich, or albitic). Intermediate rocks can have up to 25% quartz and 50% to 75% plagioclase. Mafic rocks only have plagioclase (up to 50%), and that plagioclase will be calcium-rich, or anorthitic.
Exercise: Mineral proportions in igneous rocks

Figure 3.4.3 provides a diagrammatic representation of the proportions of dark minerals in light-coloured rocks. You can use that when trying to estimate the ferromagnesian mineral content of actual rocks, and you can get some practice doing that by completing Exercise 3.6. Be warned! Geology students almost universally over-estimate the proportion of dark minerals.

Exercise 3.6 Proportions of ferromagnesian silicates
The four igneous rocks shown below have differing proportions of ferromagnesian silicates. Estimate those proportions using the diagrams in Figure 3.4.3, and then use Figure 3.4.1 to determine the likely rock name for each one.
![]() |
![]() |
![]() |
![]() |
___% | ___% | ___% | ___% |
__________ | __________ | __________ | __________ |
See Appendix 3 for Exercise 3.6 answers.
Igneous rocks are also classified according to their textures. The textures of volcanic rocks will be discussed in Chapter 4, so here we’ll only look at the different textures of intrusive igneous rocks. Almost all intrusive igneous rocks have crystals that are large enough to see with the naked eye, and we use the term phaneritic (from the Greek word phaneros meaning visible) to describe that. Typically that means they are larger than about 0.5 millitmeres (mm) — the thickness of a strong line made with a ballpoint pen. (If the crystals are too small to distinguish, which is typical of most volcanic rocks, we use the term aphanitic (from the Greek word aphanos - unseen) The intrusive rocks shown in Figure 3.3.5 are all phaneritic, as are those shown in Exercise 3.6.
In general, the size of crystals is proportional to the rate of cooling. The longer it takes for a body of magma to cool, the larger the crystals can grow. It is not uncommon to see an intrusive igneous rock with crystals up to 1 centimetre (cm) long. In some situations, especially toward the end of the cooling stage, the magma can become water rich. The presence of liquid water (still liquid at high temperatures because it is under pressure) promotes the relatively easy movement of ions, and this allows crystals to grow large, sometimes to several centimetres (Figure 3.4.4). Finally, as already described, if an igneous rock goes through a two-stage cooling process, its texture will be porphyritic (Figure 3.3.7).

Image Descriptions
Attributions
- Figure 3.4.1, 3.4.2, 3.4.3: © Steven Earle. CC BY.
- Figure 3.4.4: Pegmatite. Public domain.