2.2 Journey to the Center of the Earth
Charlene Estrada
Alfred Wegener’s Continental Drift hypothesis faced some major problems. One was that scientists of the early 1900s thought that the ocean floor was one uniformly flat basin like a cement pool. Such a flat surface beneath the ocean would not bear any evidence of past moving continents. The next chapter will address how this idea significantly changed in the scientific community. The other problem with Continental Drift was that Wegener could not convincingly explain why the continents would move.
To satisfy this issue, geologists needed to think beyond the Earth’s surface. In Wegener’s time, scientists knew some of the necessary information about the Earth’s interior; there was a crust, a mantle, and core. The key to the movement of the continents was not something happening to the crust – it was a process operating within the Earth’s interior in the mantle and the engine driving it within the core! Before we discuss these processes, it is important to understand the differences between each layer in our planet.
Layers of the Earth
In Ancient Greece, they believed that all the matter in the Universe was made up of the four basic elements: Water, Air, Earth, and Fire. Today, we have refined our understanding of the Universe: there are 118 known elements (94 of which are naturally occurring), and there are still four states of matter: solid, liquid, gas, and plasma.
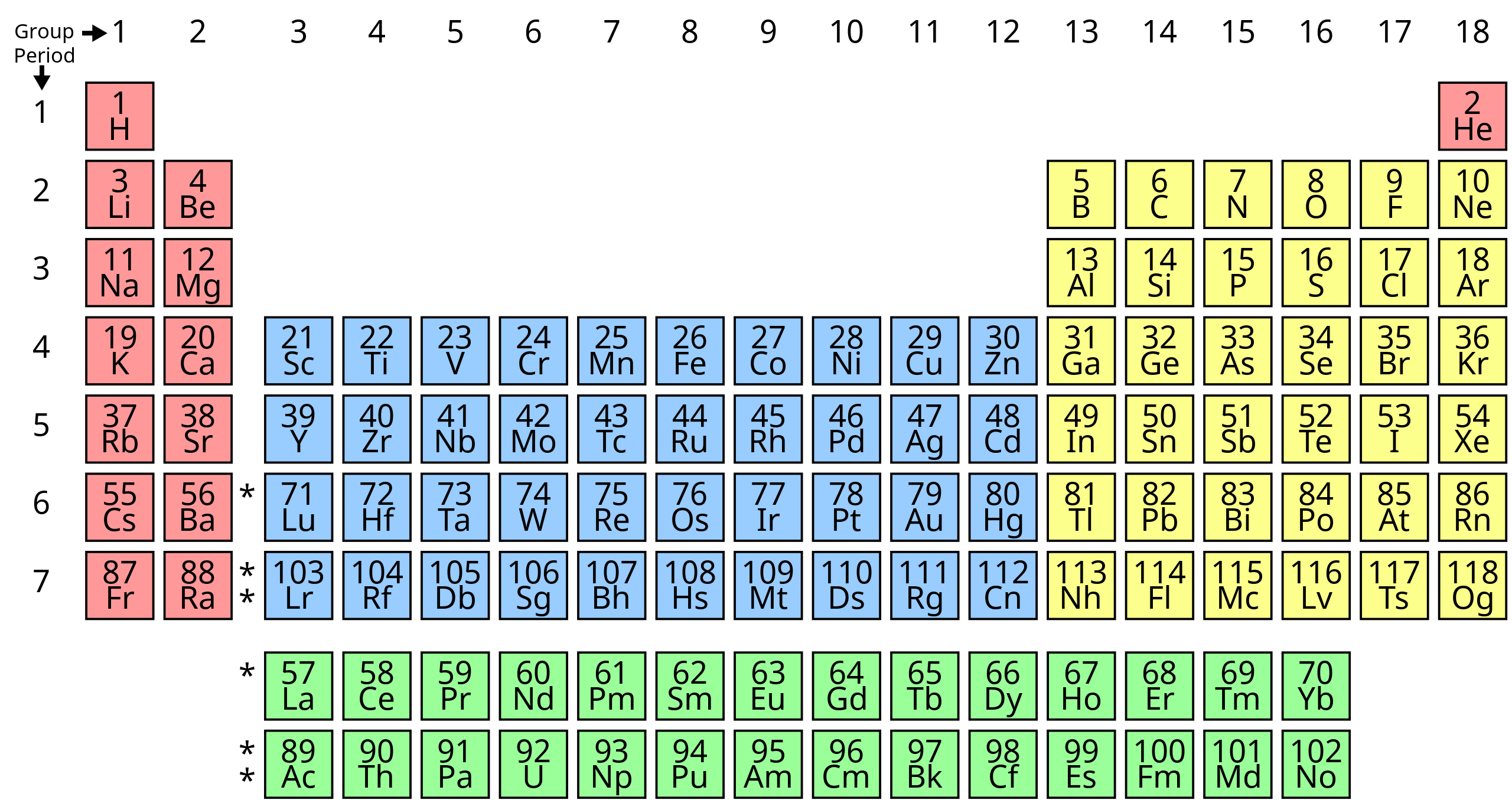
This is all-important in how we describe the interior of our planet. Our Earth is geologically active, and a big reason for that is the fact that the inside of the Earth is composed of different layers. These layers differ chemically – the bulk makeup of elements – and physically – with differences in their states of matter. All of this is a fancy way of saying that the inside of Earth is not the same as the outside!
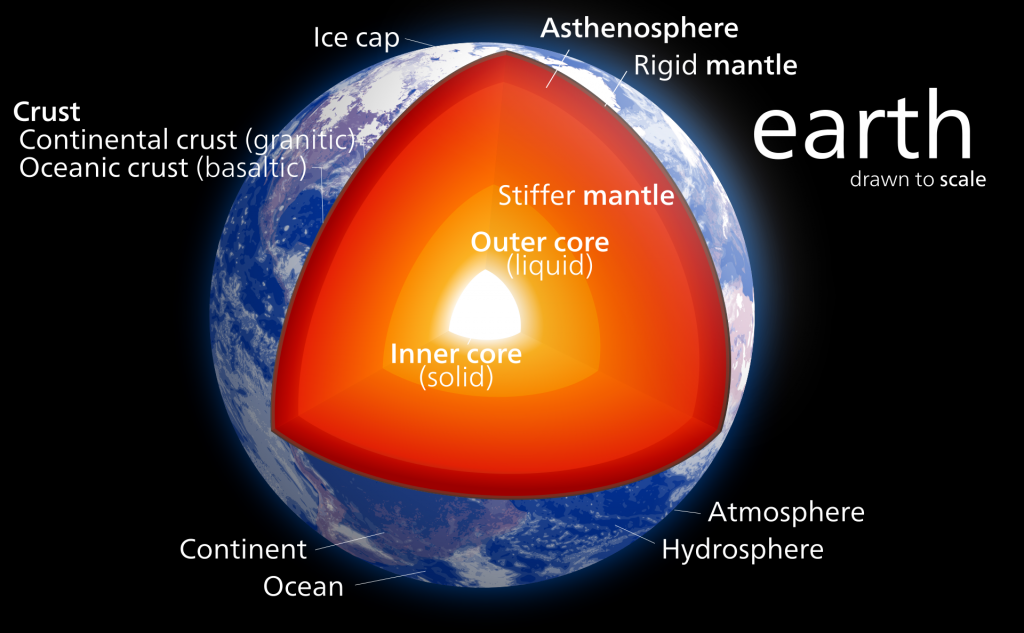
Crust, Mantle, and Core. Most of you have probably heard of the three main layers of Earth before in school, media, or elsewhere. We live on the crust. Beneath it is a hot mantle, and at the very center of the planet is a very hot core. Even if you aren’t already familiar with this basic description, there’s a good deal more to the composition of our planet. Let’s take a journey to the center of our Earth!
Video 2.2.1. Layers of the Earth mini-lecture, by Khan academy (9:32).
Or take your own journey right here!!
Stop 1: The Crust
Physical State: Solid
Chemical Composition: O, Si, Al, Fe,
Depth: 0 – 70 km (variable depending on crust type)
Temperature: 0 – 500 °C
Video 2.2.2. How much do you have to dig to reach the Earth’s core? Take a trip through markers in buried layers until you reach the center? (3:05)
The crust is where we and every known organism on the planet lives. It may be pretty important to us, but it only makes up a measly 1% of Earth’s total mass! Even if it is a very thin layer on Earth, the majority of geologic hazards we will be studying occur on the crust.
The crust is mainly composed of 4 elements: 46.6% Oxygen, 27.7% Silicon, 8.1%, Aluminum, and 5.1% Iron [7]. These elements mostly form igneous rocks in the crust, although a smaller percentage of the crust is also made of the two remaining rock types, sedimentary and metamorphic rocks. Physically, the crust is solid and pretty brittle at the uppermost regions on the surface. However, we draw some distinctions between two types of crust: oceanic and continental.
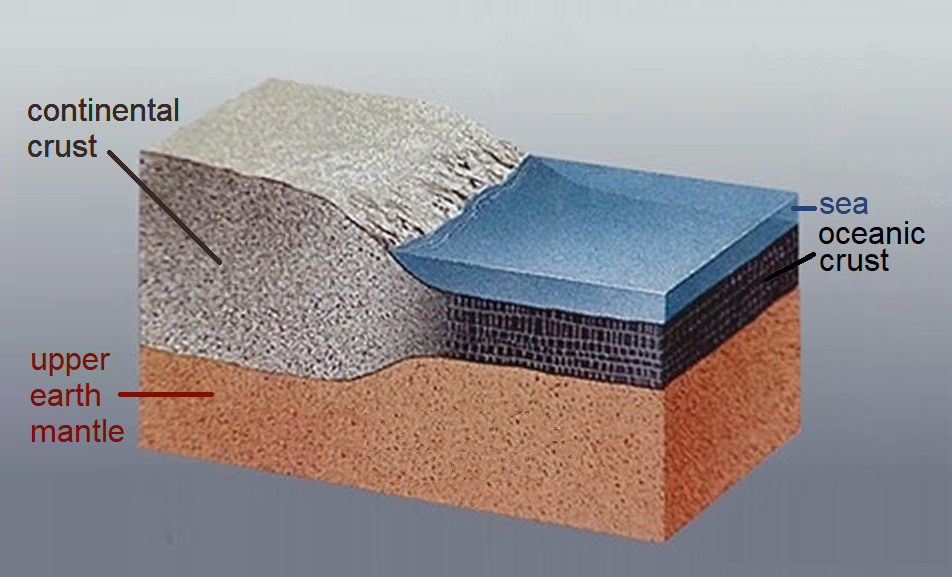
As the name implies, oceanic crust can be found underneath the world’s oceans. It is the denser of the two crust types because it contains more rocks and minerals with heavier elements such as iron (Fe). Basalt and gabbro are very common rock types found here. Oceanic crust is typically thin, about 5 km, or sometimes at maximum, 10 km, but there is another intriguing property about the crust beneath the oceans: it’s young. Although our planet is 4.54 billion years old, the oldest oceanic crust is typically about 200-300 million years old. Why is there such a disconnect? We will explore this further as we investigate plate tectonics!
The continental crust is the material that makes up the large landmasses of our world. It is lighter than oceanic crust because it contains rocks and minerals with lighter elements, such as those with more silicon and alkali elements. Some rocks that you would typically find on continental crust would be granite, rhyolite, andesite, and diorite. Unlike oceanic crust, continental crust is very thick: in some areas it can be between 40 – 70 km in depth! Continental crust can sometimes be billions of years old, and it can hold clues for the oldest events in Earth’s history.
Stop 2: The Upper Mantle
PASSPORT TO THE UPPER MANTLE
Physical State: Solid
Chemical Composition: O, Si, Mg
Depth: ~70 – 410 km
Temperature: 500 – 900 °C
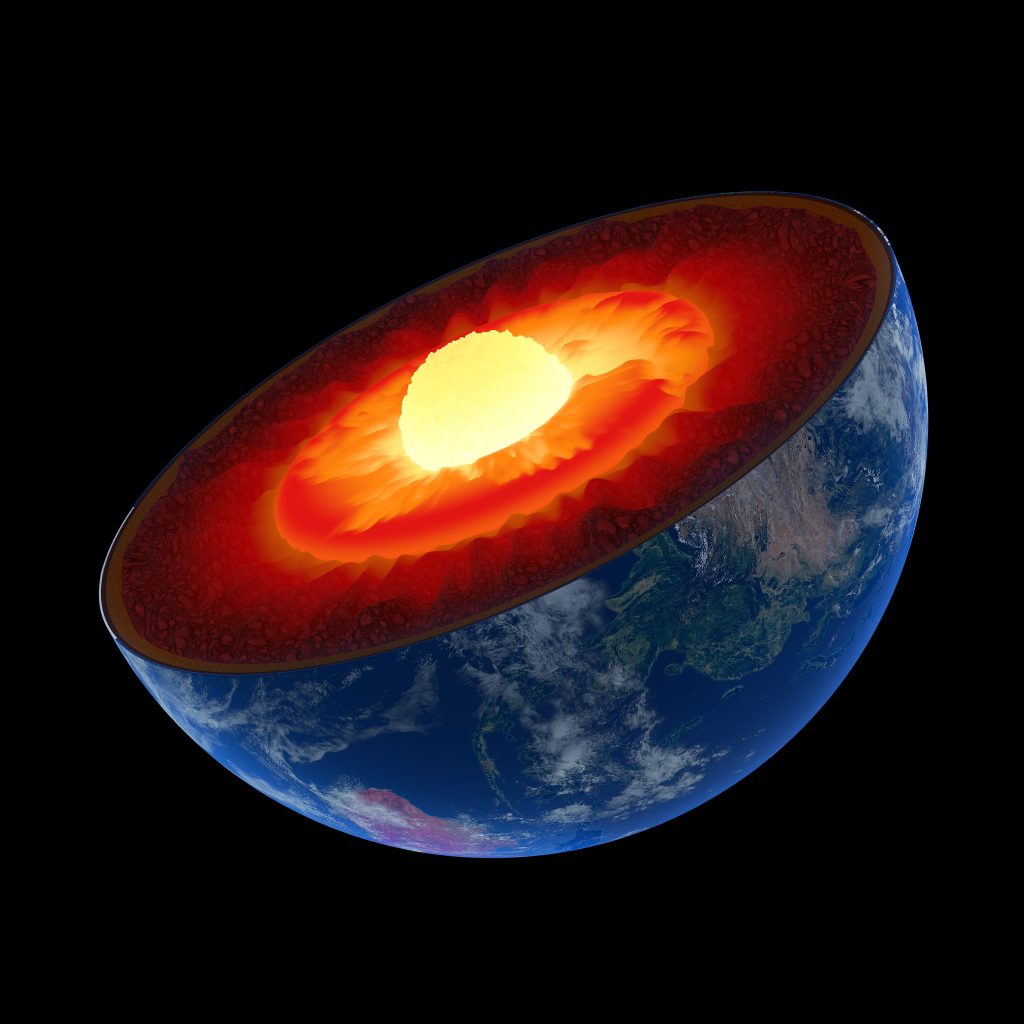
We’ve now arrived at the Earth’s mantle, which makes up over 80% of Earth’s total volume – don’t get lost! Because of its great volume and variation with increasing depth, it is more accurate to think of the mantle as being separated into two distinct zones: an upper and lower mantle. Here, we will begin with the upper mantle.
At the upper mantle, we see a distinct change in chemical composition from the crust. The mantle is much denser with an elemental breakdown of 44.8% oxygen, 22.8% magnesium, and 21.5% silicon [8]. Silicate rocks and oxide minerals often form here, but many of them would not be as common on the surface: peridotites, spinels, garnets, and olivine are all prime examples.
If you were to do a quick search for the depth of the upper mantle, you won’t get a consensus between different sources; the depth, pressure, and temperature of the upper mantle vary! The exact transition point to the lower mantle is a matter of debate because we cannot directly observe it. So, what do we know?
From the crust-mantle boundary down to about 100 km beneath the Earth’s surface, the upper mantle is rigid. This means that even though it is hotter and chemically different than the crust, this section of the mantle will tend to break and rupture when it is subjected to stress. This section of the mantle belongs to what we call the “lithosphere“, and we will discuss what that means later in the chapter.
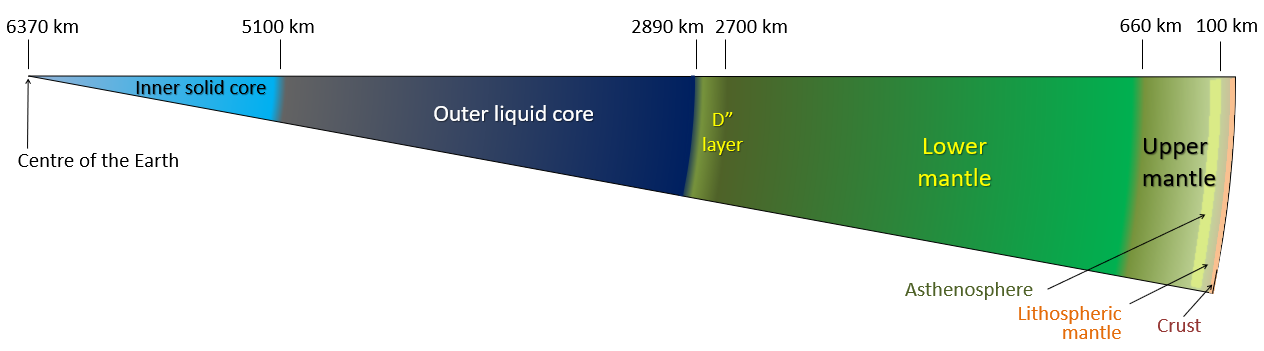
Between 100 – 410 km deep, the upper mantle becomes hotter and begins to flow and stretch easier; we call this reaction ductility or plastic flow. Nevertheless, this section of rock is still solid. This part of the mantle is called the “asthenosphere“, but don’t worry about this term and its implications just yet – all you need to know now is that with depth, the upper mantle is beginning to act differently!
Backyard Geology: San Carlos, Arizona.
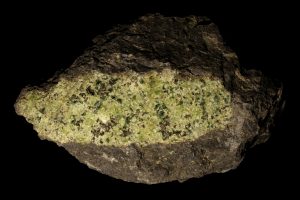
The San Carlos Apache Reservation is located east of Phoenix in Gila County. This region is a rich source of the gem peridot, which originates from the igneous rock peridotite. Peridotite is an intrusive, ultramafic rock. What makes the occurrence of peridotite and the resulting olivine/peridot unusual is that ultramafic rocks only tend to form at the mantle; we rarely find them on Earth’s surface. How did the peridotite end up at San Carlos? Up to 4 million years ago, a volcano erupted basaltic lava; however, in that eruption, it carried hardened peridotite rocks up to the surface in the process. The mafic magma would not melt the peridotite, because it originally crystallized at higher temperatures deeper in the mantle. Mantle rocks that are brought up to the surface with a volcanic eruption are called xenoliths, which imply that they did not form within or on the crust. The peridotite at San Carlos remains a literal “piece” of our upper mantle that we can physically examine – as it turns out, the mantle is actually green, not hot red or orange as most diagrams of the Earth’s interior might suggest [9]!
Pit-Stop: The Transition Zone
Around 410 – 660 km deep, we enter the transition zone. This area is wide boundary between the upper mantle and the lower mantle. There’s a lot of pressure in the transition zone, which causes the rocks there to become very dense. However, what has really fascinated scientists in recent years has been the discovery of water stored in solid minerals like ringwoodite (Mg2SiO4). We currently estimate that there’s just as much stored water in the transition zone as in our oceans [8]!
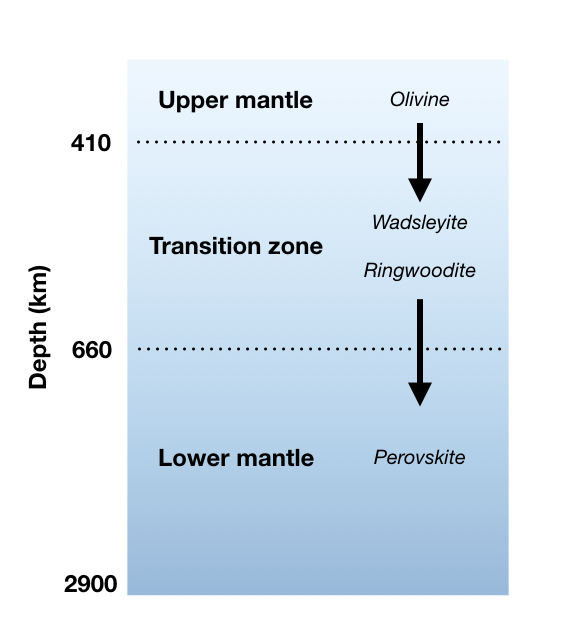
Stop 3: The Lower Mantle
PASSPORT TO THE LOWER MANTLE
Physical State: Solid
Chemical Composition: Mg, Fe, Si, O
Depth: 660 – 2900 km
Temperature: 900 – 3500 °C
The lower mantle is HUGE! It spans from 660 to 2900 km in depth, and it easily makes up the majority of the whole mantle. At up to 3500 °C, the lower mantle is very hot, but there is also plenty of pressure from the overlying layers of rocks (think over ONE MILLION TIMES the pressure we have on the surface of Earth!). Some websites and sources might claim that because of the high temperatures, the lower mantle is liquid, but this is not true! The high pressures experienced at the lower mantle keep the materials in the solid phase [8].
There’s still a lot that we don’t know about the lower mantle. It is generally agreed that because of the pressure, the rocks in this layer do not flow and stretch with ductile deformation as much as they might in the upper mantle. A lot of the lower mantle is also composed of Mg, Si, and O, but the main minerals are made of Mg, Si, and Ca, which are squeezed together in an atomic structure called the perovskite group.
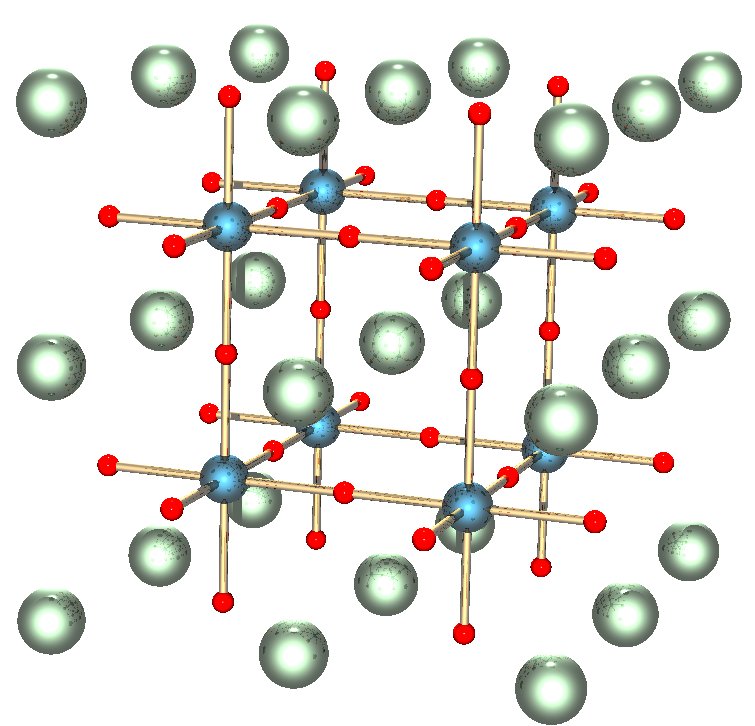
Stop 4: The Outer Core
PASSPORT TO THE OUTER CORE
Physical State: Liquid
Chemical Composition: Fe, Ni
Depth: 2890 – 5150 km
Temperature: 4,500 – 5,500 °C
Welcome to the core – it’s getting hot! At a devilish 4,500 to 5,500 °C, the material in the outer core cannot remain solid. The outer core is the only liquid layer in the Earth’s interior [10]. Just imagine – molten, liquid iron and nickel swirling around under tremendous pressures and temperatures! The outer core is not only fascinating; it is responsible for making life far more tolerable here on Earth’s surface. But how?
Iron and nickel are both metallic elements that also hold some magnetic properties – think about a refrigerator magnet attracting iron shavings, for example. When these elements move around in liquid form, they actually generate electric currents! When these currents are combined with the way the Earth is tilted slightly on its axis and rotates, a magnetic field is established.
Video 2.2.3 Why does Earth have a magnetic field? And what is the purpose that it serves? (2:04)
Why should we care about a magnetic field? Our Sun emits electromagnetic radiation that can disrupt electronics as well as destroy living tissues. These come in the form of solar flares and coronal mass ejections. Be glad we have a magnetic field around to keep us protected!
Stop 5: The Inner Core
PASSPORT TO THE INNER CORE
Physical State: Solid
Chemical Composition: Fe, Ni (possible other elements Si, C, S, ???)
Depth: 5,150 – 6370 km
Temperature: 5,200 – 6,000 °C
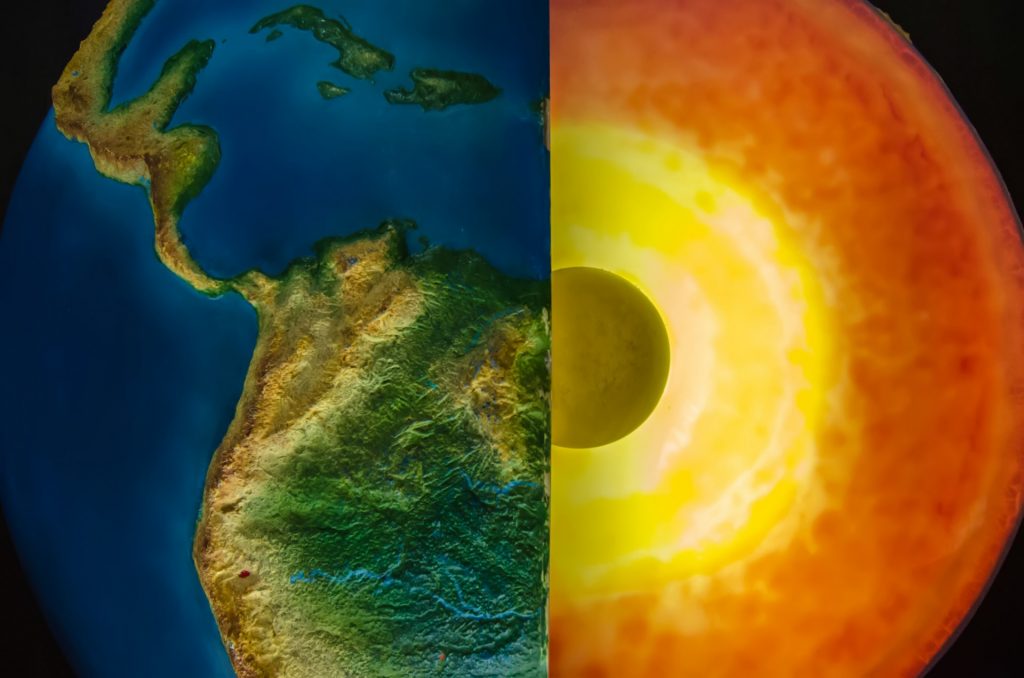
We’ve made it quite literally to the center of the Earth! Our final stop is the inner core, which is a solid sphere composed of iron and nickel. The inner core’s composition is very similar to the outer core; however, unlike our previous stop, it has remained solid! Why does the inner core remain solid while the outer core is liquid? The temperature of outer core is not much different at nearly 6000 °C, so heat is not the reason [10]. Instead, think about the other process occurring at the inner core: pressure.
To arrive at the inner core, it is necessary to travel to a depth of 5,150 km or about 3,200 miles. Under all of that densely packed rock, the inner core is subjected to at least 3.6 MILLION times the pressure we would experience at the Earth’s surface [10]! That’s enough to keep molten hot iron and nickel in solid form!
Besides iron and nickel, scientists believe that there are trace amounts of other elements in the Earth’s core. The identity of these elements has not been well agreed upon yet – they could be anything from sulfur (S) to silicon (Si) or even carbon (C)! Another strange property of the inner core is that it might even be divided further into an inner-inner core. Geologists suggest that the crystals making up the iron in the outer shell are oriented North and South, whereas those in the inner-inner core are oriented East and West (Stephenson, Tkalčić and Sambridge 2015). This idea of two layers being present within the inner core has been gaining wider acceptance as of 2015, which shows us how much we are constantly learning about Earth’s interior!
A hypothesis that claims the Earth's landforms, specifically continents, move across the oceans over tens of millions of years.
The thin, outermost layer of Earth composed of rigid rock, which is home to all known life on the planet.
A hot interior layer of solid rock between the crust and core that is capable of plastic flow. The mantle is the largest layer of Earth.
The extremely hot center layer within Earth, which is composed mainly of iron and nickel.
Rocks that crystallize from molten materials beneath the Earth surface or from volcanic processes.
rocks that cement together from weathering products, either from sediments or chemical ions in water.
Rocks that form when any type of preexisting rock is warped or transformed under elevated temperatures and pressures.
A type of rigid, thin crust made of iron and magnesium rich minerals that is found beneath the planet's oceans.
Thick crustal material mostly made of feldspar and silica rich minerals which forms the world's large landmasses.
Metals in group I: lithium, sodium, potassium, rubidium, caesium, and francium.
The outermost layer of the Earth's mantle, which contains both the lower lithosphere and upper asthenosphere. This layer is susceptible to convection currents and plastic flow.
A mineral class that is primarily composed or defined by silicon (Si) and oxygen (O).
A class of minerals with a chemical composition of a metallic element bound with oxygen.
The deeper of the two mantle layers in Earth's interior, also called the mesophere. The lower mantle is hotter and more rigid than the upper mantle.
The outer, relatively rigid layer of the Earth that is composed of crust and upper mantle.
also known as ductile flow or ductility. The tendency for very hot rock to flow like putty under stress.
A ductile but solid, hot layer in the Earth composed of the lower crust and upper mantle that flows like putty over long periods of time. It drives the movement of the rigid tectonic plates riding above.
a gemstone commonly made from the forsterite (Mg-dominated) variety of the mineral olivine
an ultramafic, coarse-grained igneous rock that forms from magma and is primarily composed of olivine and pyroxene.
coarse-grained igneous rock texture with visible crystals within the matrix.
A magnesium and iron rich rock that contains very little silica.
Originating from an iron and magnesium-rich magma/lava composition.
a piece of rock in volcanic deposits that is not from the source magma, but that was created from another process.
a region between the upper and lower mantle in which dense rocks may store water in quantities as large as the Earth's oceans.
Processes that cause a rock body to flow as a solid or otherwise deform without fracturing and faulting.
The hot, liquid layer between the inner core and mantle made of iron and nickel.
A concept which describes how magnetic force is distributed around something, such as a planet.
a spectrum of known energy emitted by any body which can range from damaging gamma radiation, x-rays, UV light, visible light, infrared, microwave, to radio radiation.
the innermost or center layer of the Earth made mostly of iron and nickel and subjected to the highest temperatures and pressures.